Abstract
Understanding the communication between nerve cells in the brain is key to understanding how the brain works. Communication between nerve cells involves chemical messages sent from one cell that get translated into electrical activity in the receiving cell. This electrical activity is the core language of nerve cells and of the entire brain. How does a chemical message released in one cell results in electrical activity in another nerve cell, and how did we discovery this? Let us dive together into the electrifying world of nerve cell communication. I will tell you about our experiments, which enabled us to find the most basic component of electrical activity in the brain—ion channels. The discovery of ion channels paved the way to understanding the origin of electrical activity in the brain and other organs like the heart. This discovery provided new insights into the development of drugs for treating various electrical-related diseases, such as epilepsy and heart-rate disorders.
Professor Bert Sakmann won the Nobel Prize in Physiology or Medicine in 1991, jointly with Erwin Neher, for their discoveries concerning the function of single ion channels in cells.
How do Cells Communicate With Each Other?
Your body and every other living body is made of cells—the basic building blocks of life. Each cell is both an individual unit, with its own independent functions, and part of the whole multicellular organism (like the brain and the heart) which needs to operate in a coordinated way. Every cell is surrounded by a clear physical border, called the cell membrane, which separates the cellular contents from the outside (extracellular) environment and from other cells. The membrane allows each cell to have a defined interior environment and to perform its own specialized functions. But, since individual cells are part of a larger structure, most cells—and especially nerve cells—must communicate with other cells. How do cells communicate with each other if they are separated by the cell-membrane barrier? Well, there are several mechanisms that allow cells to communicate with each other. One of the most common ways, and the way we will focus on here, is that a cell sends a chemical messenger substance to the receiving cell [1]. By detecting this substance, the receiving cell “knows” that a signal was sent to it from another cell, and the receiving cell responds accordingly.
Communication Between Nerve Cells
Nerve cells, the elementary building blocks of the brain, “speak” the language of electricity. At every given moment, each nerve cell shows a specific electrical activity, producing a set of brief electrical pulses called spikes. Together with other large networks of active nerve cells, a whole “electrical symphony” is generated in the brain. This electrical activity in large networks of nerve cells in our brain is correlated with every aspect of our behavior and experience; our actions, thoughts, feelings, and memories.
How do nerve cells communicate with each other to create such a coordinated “electrical symphony?” Communication between nerve cells is more complex than communication between other cell types, since it includes both chemical and electrical components. This communication happens at a specific contact location between the cells called the synapse, and is comprised of two basic steps. First, the sender cell secretes (emits) a chemical substance, called a neurotransmitter [1], into the extracellular space (a gap) between the sending and receiving cells. When the neurotransmitter arrives (via diffusion) at the receiving cell, it binds there to specific receptors and, consequently, ions start to flow across the membrane of this cell. As a result, electrical activity is generated in the receiving cell (Figure 1).
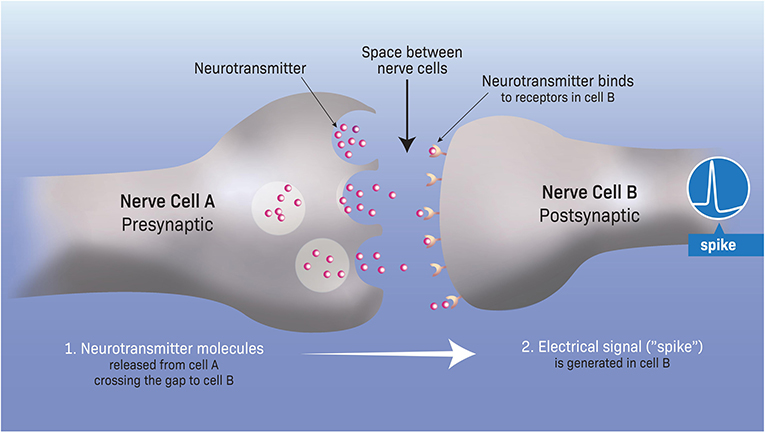
- Figure 1 - Messaging between nerve cells at the synapse.
- Communication between nerve cells happens at a specific contact location between the cells called the synapse. First, the transmitting (presynaptic) nerve cell (cell A) releases a chemical substance, called a neurotransmitter, into the small space between the cells. The neurotransmitter crosses this gap and binds to the receiving (post-synaptic) nerve cell (cell B). Consequently, ion channels open in the membrane of the post-synaptic cell through which ions start flowing, giving rise to an electrical signal called a spike (blue circle at right).
Ions and Membrane Channels in Nerve Cells
Most of the electrical currents in the brain are generated by a small group of ions—four in particular. Three of these ions are positively charged (sodium—Na+, potassium—K+, and calcium—Ca++) and one is negatively charged (chloride—Cl-). These ions can enter or exit through the membranes of nerve cells. When they do, they change the electrical potential between the two sides of the cell’s membrane. These rapid changes in the electrical potential across the nerve’s membrane underlie the generation of the basic electrical “word” (or “bit”) that nerve cells use; the electrical signals called spikes (Figure 1). You can think of a spike as a very short bolt of lightning—a brief (1 ms, which is 1/1,000 of a second) and tiny (one-tenth of a volt, or 100 millivolts) electrical pulse, that occurs in nerve cells when they are active.
How do these ions cross the membrane barrier of nerve cells to generate electrical activity? And how does the neurotransmitter released from one cell translate into electrical activity in the receiving cell? There must be some pathway for ions to cross the, otherwise insulating, membrane of the receiving cell. When I began to study this field, the mechanism by which ions move through nerve cell membranes was not clear.
Using a special experimental technique that I developed with my colleague, Prof. Erwin Neher [2], we found that ions do pass between the two sides of the membrane, according to their chemical gradients. We found that ions cross the membrane through small “holes” in the membrane, called pores. These pores are proteins that serve as channels connecting the outside and the inside of the cell. Since ions are the substances that pass through these pores, they are called ion channels (Figure 2). We found that ion channels open and close rapidly in response to neurotransmitters. The opening and closing of specific ion channels (for example, channels that are specific for Na+ ions or K+ ions) enables the flow of ions across the cell’s membrane. This current flow, in turn, changes the electrical potential across the membrane. In response, the receiving cell generates the sparky spike—the basic electrical “word” in the brain.
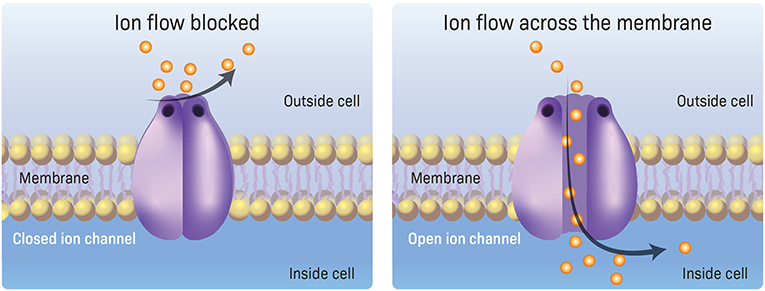
- Figure 2 - Ion channels in the nerve membrane.
- Ion channels (purple) are pores (holes) made of proteins, which are located within the membranes of nerve cells. On the receiving (post-synaptic) cell (see Figure 1) these channels are typically closed (left) but they become open (right) in response to neurotransmitters released from the presynaptic cells. The opening of membrane ion channels enables the flow of ions (orange balls) across the membrane, and this serves as the basic mechanism for generating electrical activity in nerve cells.
Discovering Ion Channels: the Patch Clamp Technique
When Prof. Neher and I started studying ion flow in nerve cells, we considered two main ion-transport mechanisms that could be at work. The first mechanism involved a transport molecule. According to this idea, specific molecules in the membrane “catch” one ion at a time, pass it from the outside of the cell to the inside, and release it there. This mechanism was known to occur in other bodily processes, such as in energy production, in which nutrient molecules pass across the cell membrane via transport molecules.
The second possible mechanism that we considered, which was later confirmed by our experiments, was that ion channels exist in the membrane for specific ions. These channels may be either open or closed. When open, current can flow between the two sides of the membrane, connecting the external environment of the cell to its interior environment (Figure 2). To examine whether this mechanism is responsible for the transport of ions into and out of cells during the generation of spikes, we had to study the electrical activity resulting from ions crossing the membrane through individual ion channels. To do this, we had to isolate a very small area, or “patch,” of the nerve cell’s membrane, hoping to measure the electrical current passing through a single ion channel that might exist in this small patch. If ion channels existed, then we would expect to measure a certain pattern of electrical activity corresponding to the opening and closing of the ion channel, which is different than the electrical pattern expected if transport molecules were used to move ions across the membrane.
To perform this kind of current measurement, we had to overcome two main challenges. First, we had to measure ions flowing across the membrane channel in the small patch of membrane without losing any of this current. This is difficult because, if the recording device is not tightly sealed to the membrane, ions that cross the membrane through the channel might escape sideways before entering the detection device. We therefore needed to make sure that the ions crossing the membrane were forced to flow thorough the detector.
The second challenge was to discriminate between two types of currents that flow through the nerve cell membrane. As it turns out, the membrane is always electrically active—a phenomenon that is called background noise. Background noise appears as constant electrical activity that is different from the electrical activity related to the flow of ions through the membrane in response to neurotransmitters. The background noise can be very large compared to the current that we wanted to measure from the opening of individual membrane channels. Therefore, we had to find a way to reduce the background noise so that it would not overpower (or “mask”) the current flowing through single ion channels.
We solved both challenges by using a very thin glass tube called a pipette, with a tip around one micrometer (1/1,000 of a millimeter) in diameter (Figure 3A). The other end of the pipette has an amperemeter, which measures electrical current. The tip of the pipette is strongly pressed against a small patch of the cell’s membrane and suction is applied, creating a very tight contact between the pipette tip and the membrane to ensure that no leakage of ions can occur. Recordings from such a small patch of membrane also reduced background noise and, therefore, improved the recording of the flow of ions through the ion channel.
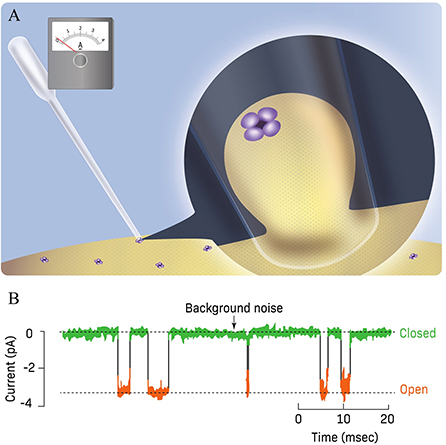
- Figure 3 - Current flow through membrane ion channels.
- (A) The patch clamp technique. The tip of a thin glass pipette is tightly sealed against a small patch of the cell membrane containing an ion channel (purple, see enlarged version at right). The pipette contains neurotransmitter that binds to the membrane and open an ion channel, which enables ions to flow across the membrane. The current flowing through the ion channel is measured by an amperemeter connected to the pipette. (B) Measurement of current flowing through a single ion channel in a small membrane patch. This ion channel opens and closes spontaneously in response to the binding (open) and unbinding (closed) of the neurotransmitter to the membrane receptor (Figure 1). When the ion channel is closed, a noisy background current is measured (green). When the ion channel opens, a rapid downwards, step-like, current is observed (orange) (Figure adapted from Neher and Sakmann [2]).
Current Flow Through the ion Channel
When no neurotransmitter was present, we found that no current passed through the channel and only minor background noise was observed (Figure 3B). When a neurotransmitter bounds to the membrane, the ion channel opened very rapidly, in a step-like manner, enabling the flow of a tiny current of a few picoamperes1 across the membrane [2–4]. The unbinding of the neurotransmitter caused the channel to close again (Figure 3B). We found that the ion channel stayed open or closed for only milliseconds (a millisecond is 1/1,000 of a second) and that both the duration of the open state and the time interval between openings of the channel varied, due to the sporadic binding of neurotransmitter molecules. As you can see in Figure 3B, the amplitude of the current when it flowed through the open channel was quite constant.
After measuring the tiny current that flowed through the membrane patch and making some calculations, we estimated that around 10,000 ions crossed the small patch of membrane every millisecond. This told us that the opening of ion channels, rather than the transport of ions via transport molecules, is the mechanism enabling ions to across the cell’s membrane! Transport molecules are too slow to transport ions across the membrane at such a high rate. This was an important discovery, as it confirmed the existence and function of ion channels as the basic mechanism producing the electrical activity, including the spike, in nerve cells. These ion channels are also responsible for the generation of electrical activity in other “excitable” tissues, such as peripheral muscles and the heart.
Furthermore, it was important to understand the functioning of membrane ion channels because many neurological disorders (as well as disorders of the heart and other body tissues) are due to ion-channel dysfunctions. Consequently, the new term “channelopathies2” was coined to describe the (very large) family of diseases caused by defects in the functioning of ion channels. Based on the discovery of membrane ion channels and their function, my colleague Prof. Erwin Neher and I were awarded in 1991 the Nobel Prize in Medicine or Physiology.
Recommendations for Young Minds
I will start by telling you the most important thing I have learned from my scientific supervisor, Prof. Bernard Katz, who also received the Nobel Prize in Physiology or Medicine in 1970. He taught me that you need to be very critical about your results and always be ready for new findings that might invalidate your previous findings—as unpleasant as that might be. I try to pass this lesson on to my students and teach them to be critical of their results. Especially in biological tissues, there are many influences we cannot control and that must be taken into consideration. Therefore, when my students have a new finding, I advise them to keep it to themselves for a while and repeat their experiments to try to invalidate their results over and over again. They should only publish their results once they are completely convinced those results are correct.
From a more general view of life, in my opinion a good life is one in which you have something to think about that gives you the opportunity to follow your curiosity and possibly discover something new. In other peoples’ perspectives, a good life could mean earning a lot of money or being recognized by others, and that is completely fine as well. I think that being a scientist is the best choice you can make, but only if you are curious about Nature. Do not try to be a scientist because you think it is a glamorous profession. If your insides do not burn with the urge to find things out, it is better to choose another profession—one that does make you burn with excitement and passion.
Additional Materials
Glossary
Nerve Cells: ↑ The major cellular building-blocks of the brain. Nerve cells generate the electrical activity of the brain.
Synapse: ↑ A contact point between two nerve cells, consisting of a small gap where chemical substance—the neurotransmitter—passes from the sending cell (the presynaptic cell) to a receiving cell (the post-synaptic cell).
Neurotransmitter: ↑ A chemical substance that is released from one nerve cell and received by another nerve cell, enabling communication between nerve cells.
Diffusion: ↑ A process of spontaneous movement through which particles move undirected from one place to another.
Ion: ↑ A particle with either a positive or negative charge.
Electrical Potential: ↑ A difference in charge between two points, in our case between the two sides of the membrane. Ions with positive charge will flow from the more positive side to the less positive side.
Chemical Gradient: ↑ A difference in the concentration of a substance across locations. The substances, in our case ions in the two sides of the membrane, move “down” the gradient, from the side with higher concentration to the side with lower concentration.
Ion Channel: ↑ A small hole (pore) made of proteins in the cell’s membrane which, when open, enables the passage of ions into and out of the cell.
Conflict of Interest
The author declares that the research was conducted in the absence of any commercial or financial relationships that could be construed as a potential conflict of interest.
Acknowledgments
I wish to thank Noa Segev for conducting the interview which served as the basis for this paper, and for co-authoring the paper.
Footnotes
1. ↑Picoamperes (pA) are used to measure very tiny electrical currents—one pA is 10-12 amperes.
2. ↑See here on groups of diseases caused by the disfunction of ion channels https://en.wikipedia.org/wiki/Channelopathy.
References
[1] ↑ Katz, B. 1971. Quantal mechanism of neural transmitter release. Science. 173:123–6.
[2] ↑ Neher, E., and Sakmann, B. 1992. The patch clamp technique. Sci. Am. 266:44–51.
[3] ↑ Hamill, O. P., and Sakmann, B. 1981. Multiple conductance states of single acetylcholine receptor channels in embryonic muscle cells. Nature. 294:462–4.
[4] ↑ Bormann, J., Hamill, O. P., and Sakmann, B. 1987. Mechanism of anion permeation through channels gated by glycine and gamma-aminobutyric acid in mouse cultured spinal neurones. J. Physiol. 385:243–86.