Abstract
The brain is the control center for all our thoughts and actions. Have you ever wondered exactly how your brain works as you run, jump, and play? To look inside the working brain, scientists use a combination of high-tech microscopes, lasers, and genetic engineering, which allows them to directly see the cells within the brain—called neurons. Scientists can make neurons glow when those cells become active, which tells scientists the neurons are passing information to each other in large networks. In this way, neurons take in information from the environment and use it to plan your next move. We will discuss the biology, physics, and virtual tools that scientists are using to directly examine the activity of neurons in the brains of mice. The findings from these experiments help scientists understand how neurons work together, how they help us learn and remember, and what goes wrong in disorders that affect the brain.
Biology: Neurons, Networks, and Activity
The human brain consists of many tiny structures called cells. The most common cell type in the brain is called the neuron. Neurons are like little machines that keep the brain running and allow us to see, to think, to plan our movements, and even to read this sentence! Our brains have over 86 billion neurons—so many parts working together to allow us to interact with the world around us. Neurons form connections with each other so that they can communicate and pass important information to their neighbors. These connections create complex networks, each with specific functions. For example, to recognize a flower, first you see the flower with your eyes, then certain neurons in your brain process information about its shape and color, and these neurons share this information through a network with other neurons that process additional information about how the flower smells. Neurons within memory networks examine whether you have seen this flower before. Together, these neurons form a recognition network and allow you to determine that the flower is a daisy, for example. Likewise, there are networks that guide you through the world like a map, that help you run and jump, and that let you figure out that 2 + 2 = 4!
Neurons can pass on information using the strength and timing of small electrical currents flowing through them. To create these electrical currents, neurons use electrically charged ions, which are some of the smallest and most basic building blocks in the universe. One important ion for neurons is calcium, which can flow into the neuron through tiny openings called ion channels. When enough calcium and other ions flow into the neuron, we say the neuron is “activated”—like when you turn on a light. Activated neurons can pass chemical messages to other neurons through small contacts between them, called synapses (Figure 1). One chemical message may tell the next neuron to get to work passing on more messages, faster! This gets the other neurons very excited and produces more electrical activity. Another chemical message may tell the same neuron to stop what it is doing; this inhibits the electrical activity, and the neuron becomes quiet for a while. All this message-passing activity is happening constantly in your brain—neurons are a very active bunch. To see when neurons are activated, scientists use small molecules that can sense calcium ions and then start to glow—these are called calcium sensors (Figure 1) [1]. One way to get calcium sensors into a neuron is by using genetic engineering to alter the DNA. Since a calcium sensor is made up of several proteins, scientists can add the blueprints of these proteins to an animal’s DNA, to create mice in which calcium sensors are present in specific types of neurons. These mice are otherwise just like normal mice, except the calcium sensor in each neuron has a green fluorescent protein that can glow when it binds to calcium ions, if a bit of energy is provided by shining laser light onto the neurons. The glowing complex first absorbs the energy from the light and then quickly loses this energy by emitting light of its own—hence the glow. When the neuron is activated, this glowing process happens very quickly, within a fraction of a second, and lasts until the neuron becomes inactive again. Once the neuron is inactive, it becomes dark and is no longer visible. By watching the changes in glowing neurons over time, we can follow the communications of neurons within their networks.
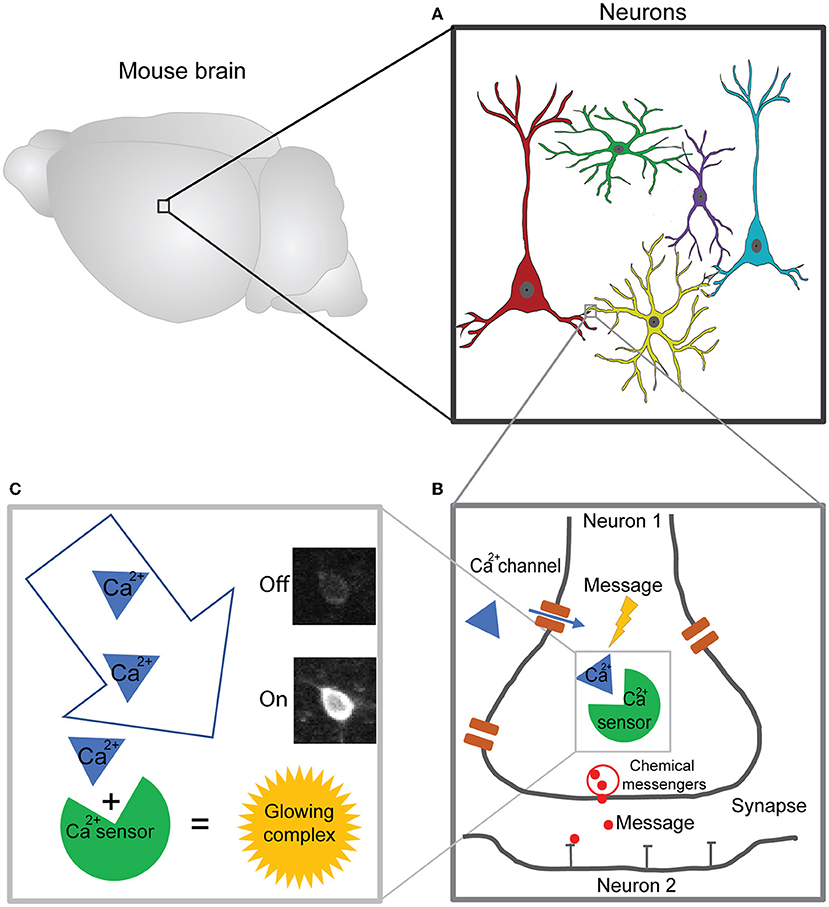
- Figure 1 - Visualizing the activity of neurons in the brain using calcium sensors.
- (A) The brain consists of neurons. (B) The activity of neurons depends on the flow of calcium and other ions, which generates chemical messages that are sent between synapses. (C) Scientists can track the concentration of calcium ions within a neuron using the “glow” produced when calcium binds to a calcium sensor. The glow means the cell is “on” or active and can pass messages to other neurons in its network.
Physics: The Two-Photon Microscope
To directly observe the glowing neurons in the brain, we use a two-photon microscope. There are three important technical details that make looking directly into the brain possible (Figure 2). First, a two-photon microscope has a special laser, and its laser beam provides the energy for the calcium sensors to glow after they bind to calcium ions. The laser beam is directed by mirrors and glass lenses toward the second most important part of the microscope: the objective. The objective magnifies the tiny neurons, which are each around 10 μm in size (0.01 mm). The magnification is usually around 20 times higher than that of the naked eye. The objective focuses the laser beam into the mouse brain, enabling the calcium sensor to glow, and it also collects the emitted (glowing) light and directs it to the third most important microscope part: the detector. The detector is called a photomultiplier tube, and it amplifies the emitted light from the neurons and converts it to a signal, like a live camera. Once we make a small glass window into the brain of the mouse, we can watch a video of the activated neurons flashing on and off via a connected computer screen, and we can also record them. We can analyze these videos to get a signal for each neuron over time, so we can understand the neuron’s activity pattern and how neurons are communicating within their networks.
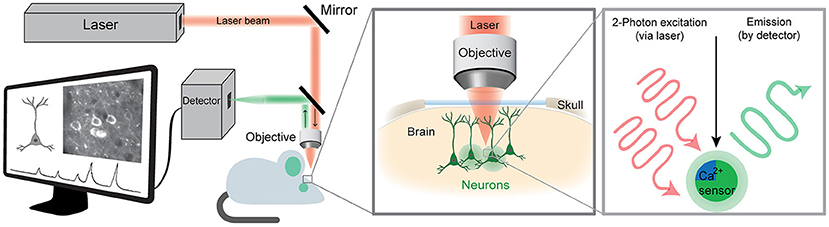
- Figure 2 - Imaging brain activity using a two-photon microscope.
- The laser light (shown in red) passes through several mirrors and lenses within the microscope to excite the calcium sensor (excitation). If the neuron is active and therefore contains a lot of calcium ions, the light emitted (emission) is bright enough (shown in green) to be seen by the detector and can be analyzed using a computer. The pattern of neuronal activity is assessed over time, creating a readout of high and low activity for each neuron as the mouse is performing various behaviors.
Behavior: Navigating in a Virtual Environment
This technique, called two-photon calcium imaging, allows us to observe what is happening in the brains of mice while they perform certain tasks [2]. Such experiments could help us to understand what might be going wrong in certain brain disorders, and it can also help us understand the role that specific neurons play in various behaviors. For example, one task in our experiments is for mice to navigate through a maze (Figure 3A). This maze is part of a virtual reality system that the animals control with their movements. It is basically a tiny cinema for the mice, made up of several monitors surrounding the animal. To move and navigate through the maze, the mice must use a running ball as a controller, just like running on a treadmill. The running ball floats on pressurized air so that the animal can run forward, backward, left, right, straight, or in circles. The movement of the ball is processed by a computer, which then translates this movement into the mouse’s position within the virtual maze [3]. So, just like in a virtual reality video game, as the mouse moves, it navigates through the virtual environment.
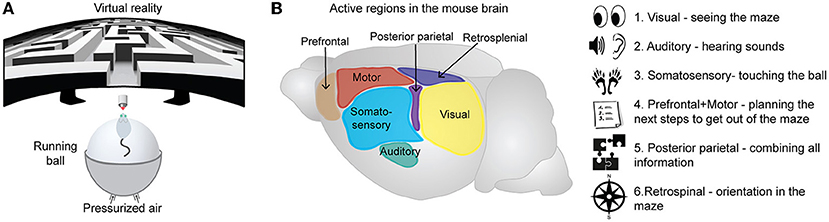
- Figure 3 - Specific brain regions help mice to find their way through a virtual maze.
- (A) Mice navigate by running on a ball pushed up by pressurized air. The virtual environment is controlled by the movement of the mouse on the running ball. (B) Regions of the mouse brain, specifically of the cerebral cortex, that may contain activated neurons during the virtual task, including sensory (visual, auditory, somatosensory), motor, and association regions (retrosplenial, prefrontal, and posterior parietal) of the cortex.
How Neurons Work Together
To find their way out of the maze, the mice must use certain regions in their brains to perceive the environment and navigate through it (Figure 3B) [4]. First, neurons of the visual system are activated so they can see the maze, the colors, and the edges of the walls. The main brain region for visual information processing is the primary visual cortex. If there are sounds in the virtual maze, neurons in the auditory cortex are involved. The sensation of touching the ball as the mouse is running through the maze is mediated by the somatosensory cortex. Second, neurons that are activated for the planning, controlling, and performance of the animal’s actual movements are located in the motor cortex and prefrontal cortex. All this sensory and motor information must also be combined, and this involves neurons in association regions, such as the posterior-parietal cortex. Finally, for the mouse to know exactly where it is in the maze, neurons in regions like the retrosplenial cortex will be activated [5]. Therefore, sensory, motor, and association networks all work together, passing messages back and forth to each other continuously so that the mouse can find its way through the maze. Humans also use the same general sensory, motor, and association brain networks to navigate their way through the real world every day.
Conclusion
Using two-photon imaging and related techniques, scientists can literally watch the activity of neurons and neuronal networks in the brain while animals are in action. The biology, physics, and experimental design behind this approach are complex, but so is the brain! Using detailed analysis of the neuronal activity patterns collected using these techniques, scientists can investigate a variety of questions about how the brain works. For example: “How do we navigate through our environments?”, “What is the contribution of each of our senses?”, “How are memories of the environment formed in these networks?” and “How do illnesses that affect neurons disrupt these networks?” These questions also include aspects of learning, forgetting, attention, aging, and disease—all important in our daily lives and all controlled by the billions of tiny neurons in our brains, working together.
Glossary
Neuron: ↑ A type of cell found in the brain; they are the basic units that make up the nervous system.
Ion: ↑ Is a positive or negative electrically charged molecule.
Ion Channel: ↑ Is like a little door or tunnel made of proteins that forms in the outer wall of a cell and is used to transport ions in and out of the cell.
Synapse: ↑ Specialized gap that forms a connection between two neurons. Synapses allow neurons to pass signals or messages to one another, which allows our brain to process information.
Calcium Sensor: ↑ A group of special proteins that scientists have engineered to glow when calcium ions are nearby within a cell.
Genetic Engineering: ↑ Is a process of changing the genes (tiny units of information) of an organism.
Two-Photon Microscope: ↑ A special microscope that uses photons (“packets” of laser light) to see deep into living brain tissue.
Acknowledgments
This work was funded by the European Regional Development Fund (ERDF: Center for Behavioral Brain Sciences ZS/2016/04/ 78113).
Conflict of Interest
The authors declare that the research was conducted in the absence of any commercial or financial relationships that could be construed as a potential conflict of interest.
References
[1] ↑ Chen, T. W., Wardill, T. J., Sun, Y., Pulver, S. R., Renninger, S. L., Baohan, A., et al. 2013. Ultrasensitive fluorescent proteins for imaging neuronal activity. Nature 499:295–300. doi: 10.1038/nature12354
[2] ↑ Dombeck, D. A., Khabbaz, A. N., Collman, F., Adelman, T. L., and Tank, D. W. 2007. Imaging large-scale neural activity with cellular resolution in awake, mobile mice. Neuron 56:43–57. doi: 10.1016/J.NEURON.2007.08.003
[3] ↑ Henschke, J. U., and Pakan, J. M. P. 2020. Disynaptic cerebrocerebellar pathways originating from multiple functionally distinct cortical areas. Elife 9:1–27. doi: 10.7554/ELIFE.59148
[4] ↑ Wolbers, T., Hegarty, M., Büchel, C., and Loomis, J. M. 2008. Spatial updating: how the brain keeps track of changing object locations during observer motion. Nat. Neurosci. 11:1223–1230. doi: 10.1038/nn.2189
[5] ↑ Sun, W., Choi, I., Stoyanov, S., Senkov, O., Ponimaskin, E., Winter, Y., et al. 2021. Context value updating and multidimensional neuronal encoding in the retrosplenial cortex. Nat. Commun. 12:1–17. doi: 10.1038/s41467-021-26301-z