Abstract
This article is based on an interview between the two authors.
Grid cells are special brain cells that play a key role in the brain’s navigation system. Research on these cells is one of the most interesting and rapidly advancing topics in brain science today. Much has changed since my colleagues and I discovered grid cells in 2005, and even since we were awarded the Nobel Prize in 2014. In this article, I will describe the advancements that transformed the field of grid cell research and tell you about the way that we study grid cells today. Finally, I will give you a peek into how we hope to use our understanding of grid cells as a “window” to understanding the brain as a whole.
This article assumes readers have the basic knowledge shared in previous articles on grid cells and place cells.
Professor Edvard Moser won the Nobel Prize in Physiology or Medicine in 2014, jointly with Prof. May-Britt Moser and Prof. John O’Keefe, for their discoveries of cells that constitute a positioning system in the brain.
Grid Cells—A Short Recap
Have you ever wondered how your brain maps the world so you can navigate successfully in it? As you might have read in a previous Nobel Collection article, grid cells are a specific type of neurons (brain cells) that are an essential part of the brain’s navigation system. Grid cells were found by my colleagues and I in 2005 [1]. They are located in a brain region called the entorhinal cortex. Each grid cell responds to a specific hexagonal pattern of locations (Figure 1). The joint activity of many grid cells creates an internal “coordinate system” in the brain that helps an animal know where it is in the environment, how to get from one location to another, and how to estimate the distance between points (to learn more about grid cells, you can watch this video).
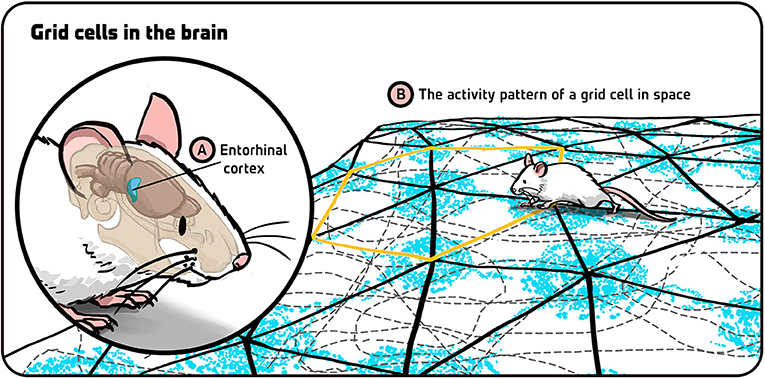
- Figure 1 - Grid cells in the brain.
- (A) Grid cells are found in a part of the brain called the entorhinal cortex. (B) When an animal moves around in its environment (gray dotted lines representing the animal’s movement in space), each of its grid cells is active at specific locations (blue dots, representing the activity of one specific grid cell). The activity pattern of each grid cell creates a hexagonal, grid-like pattern in space (yellow hexagon highlighting one such hexagon within the grid pattern). Illustration by: Iris Gat.
Grid cells work in coordination with other types of navigation cells in the entorhinal cortex, including head-direction cells, object-vector cells and border cells. Grid cells also work in coordination with place cells, which are located in another region of the brain called the hippocampus [2]. Our aim is to understand how groups of grid cells work within themselves and how they work with those other cells as well.
Current Research in Grid Cells
Since our discovery, important and rapid changes have taken place in the field of grid cell research. In 2005, the activity of grid cells was studied by examining the electrical activity of one grid cell at a time, which allowed us to discover the unique hexagonal activity pattern of grid cells. In the past few years, however, the single-cell approach was replaced by the study of the activity of many grid cells at the same time. These days, scientists who study grid cells examine the activity of networks of grid cells and how these networks represent an animal’s environment. But how can we keep track of many grid cells that are active simultaneously?
This new approach was enabled by two technologies. The first was the development of new electrodes called Neuropixels probes that enable us to “listen to” neurons by recording the electrical activity of many of them simultaneously (Figure 2A) [3, 4]. The most recent Neuropixels probes contain more than 5,000 recording sites that can pick up electrical signals created by individual neurons. Using Neuropixels probes, scientists can record the electrical activity of whole groups, or populations, of neurons—that is why this type of recording is called a neural population recording. Currently, we can record from about 380 sites on a microchip at once, giving us access to the activity of more than a thousand cells at the same time. I expect that by 2025 we will be able to record from most or all possible sites at once, which will increase the cell numbers by another order of magnitude.
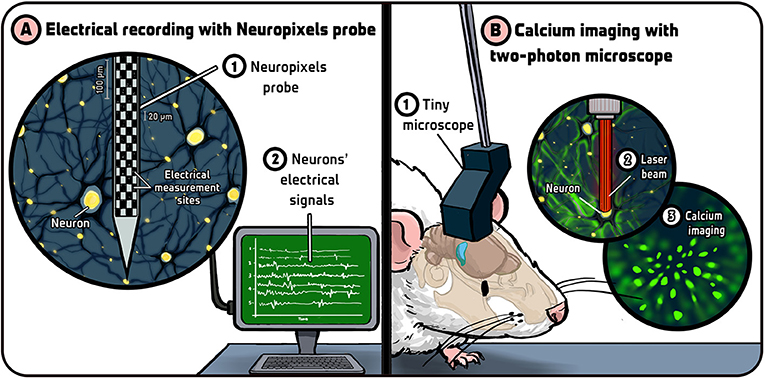
- Figure 2 - Technological developments in grid cell research.
- (A) Recently, a new device for measuring the electrical activity of many neurons simultaneously was developed, called a Neuropixels probe (1). This device is essentially a small computer chip with many recording sites (black squares) from which it can measure brain activity (2). (B) A tiny two-photon microscope is placed on the animal’s head (1). It shines a laser beam on neurons (2). The calcium inside the neurons then fluoresces in green, allowing scientists to see active neurons in a specific brain area (3). Illustration by: Iris Gat.
The second recent technological advancement was the development of exceptionally light microscopes that can be placed over the animal’s head [5]. These are miniature two-photon microscopes that can pick up changes in the flow of calcium (Ca2+) ions within neurons (Figure 2B) and tell us which cells are active at any given moment. Using calcium imaging, we can “see” grid cells by having a visual image of the active cells in the network, and combine different images to create a video of active cells over time (Video 1) [6].
- Video 1 - Calcium imaging using a two-photon microscope. Using a tiny two-photon microscope, we can track the calcium activity in the brain of an animal (bright spots on the left side of the screen) while it moves freely in its environment (right side of the screen). This tracking allows us to see which grid cells in the entorhinal cortex are active at any given moment [Video adapted from [6]].
My lab in Norway applies the advanced technologies described above to study grid cell networks. We use Neuropixels probes to record the activity of many cells in the entorhinal cortex simultaneously. Using our knowledge of grid cells, we are able to isolate the grid cells from our recordings and study them in small groups. We then ask whether certain groups of grid cells act together in a synchronized way, or whether certain groups of cells tend to be active in a specific order or arrangement when the animal moves around in its environment. If we do find such “rules” in our data, we can use them to better understand the activity of the entire network of grid cells (using advanced statistical methods and machine learning techniques). We can compare our new data to existing theoretical models of grid cell activity [7]. If we find that a certain model is accurate, we can then plan which future experiments to perform, which new experimental data to collect, and how to better analyze our data. This is the ideal scenario in science—when experiments and theory go hand-in-hand.
The Future of Grid Cells Research
There are many unanswered questions in grid cell research, such as: How do grid cells produce the activity patterns that they have? How do sub-networks (modules) of grid cells work together? And how do networks of grid cells work with networks of other types of navigation-related cells to create the brain’s complete internal map? I believe that, as we improve our understating of how various cell types operate, we will gradually be able to address the bigger question of how all these cells work together. Our next challenge will then be to understand how this collective activity leads to an animal’s perception of space—how does the activity of all these cells create the experience of navigating successfully in the world?
Another question that grid cell researchers are interested in is whether grid cells are involved in navigation planning. In other words, can grid cells predict or plan the next location(s) of the animal? And if so, from how far away? There is some indication that grid cells contain information about the immediate future location of the animal. But, if an animal is navigating a maze, then it needs to make a plan of how to get from the starting point to the end of the maze (where it gets a tasty treat). Does it make that plan right from the beginning and remember a trajectory through the maze (Figure 3A) or does it navigate “on the fly” and decide locally where to turn in each junction (Figure 3B)?
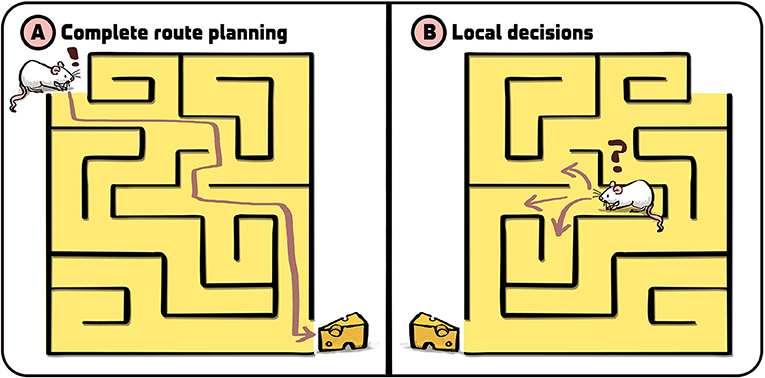
- Figure 3 - How do grid cells “plan”?
- By studying grid cells, scientists hope to find out whether animals navigate by (A) planning their whole route from point A to point B, or (B) whether they make local decisions “on the fly” at every junction along the route. Illustration by: Iris Gat.
Another direction that grid cell researchers are currently studying is whether the brain uses grid cells for other things that it needs to measure, which we call metrics [8]. Scientists speculate that grid cells are used for many types of metrics, like keeping track of social networks and understanding the “social distances” within them. In general, we think of grid cells as a neural network that performs computations in the brain. We hope to use this network, and its relations with other networks of cells in the navigation system, to understand general principles of how the brain performs computations and processes information. We believe that the grid cell system can serve as a “window” for understanding how large neural networks operate in the brain and eventually explain how cognition works—which I find very exciting!
Recommendations for Young Minds
To be a good scientist these days, the most important quality is curiosity (Figure 4). If you feel inspired to understand something, follow your curiosity and your passion—then do your best to figure it out. Do not worry about things like earning a lot of money or trying to figure out in advance what will be useful in your future. I also think it is important to be ambitious, to have high goals, and to be motivated to really make a difference for humanity. Aspire to achieve something big and, if you find it is not currently possible, break the question down into smaller steps and pursue them individually. I think that curiosity and ambition can very much be cultivated, so it is important to train yourself by facing meaningful challenges and problems.
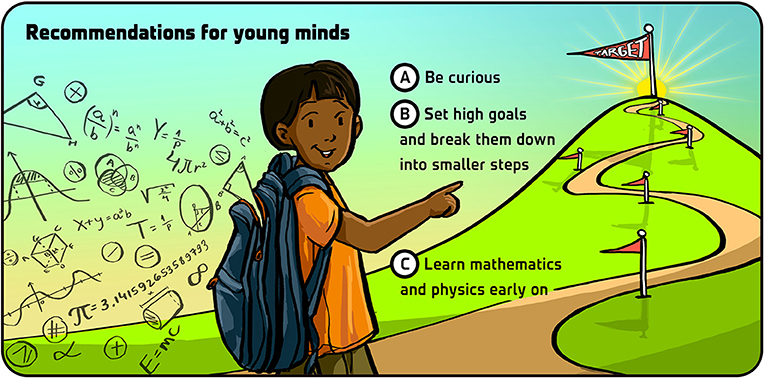
- Figure 4 - Three recommendations for young minds.
- Illustration by: Iris Gat.
If you would like to go into neuroscience, I recommend getting a solid foundation in physics and mathematics. With time, these disciplines are becoming increasingly essential for neuroscience, and they are much easier to learn at a young age than later on in your career. You will also need to know about biology, psychology, and other topics, but these are easier to learn at a later stage. Much has changed since I started my career in neuroscience in the 1980s, and I think that much more change will happen during the next 40 years. This will likely be true not only for neuroscience, but for all scientific fields. I think this makes a career in science a really exciting and rewarding adventure.
Additional Materials
- The Brain’s GPS Tells You Where You Are and Where You’ve Come from–Scientific American
- How the Mosers discovered grid cells–Kavli Institute for Systems Neuroscience
Glossary
Grid Cells: ↑ Neurons in a part of the brain called the entorhinal cortex, whose firing locations form a system of coordinates in the brain that helps an animal navigate through its environment.
Neurons: ↑ Nerve cells; cells in the brain that produce electrical impulses to send signals to the body, brain, or other neurons.
Entorhinal Cortex: ↑ A brain region containing navigation cells, including grid cells, head-direction cells, object-vector cells and border cells.
Electrodes: ↑ Measuring devices used to record the electrical activity of neurons.
Neural Population Recording: ↑ Recording of the electrical activity of many neurons simultaneously.
Metrics: ↑ Measures that supply quantitative information about some parameter (distance, in our case).
Cognition: ↑ Mental processes like thinking and remembering used to acquire knowledge and understanding.
Acknowledgments
We wish to thank Iris Gat for providing the figures. The work is supported by a Synergy Grant to E.I.M. and Yoram Burak from the European Research Council (‘KILONEURONS’, Grant Agreement N° 951319), a Centre of Excellence scheme grant to May-Britt Moser and E.I.M. from the Research Council of Norway (grant number 223262), the Kavli Foundation (May-Britt Moser and E.I.M.), and a direct contribution to May-Britt Moser and E.I.M. from the Ministry of Education and Research of Norway.
Conflict of Interest
NS declared that they were an employee of Frontiers, at the time of submission. This had no impact on the peer review process and the final decision.
The remaining author declares that the research was conducted in the absence of any commercial or financial relationships that could be construed as a potential conflict of interest.
References
[1] ↑ Hafting, T., Fyhn, M., Molden, S., Moser, M. B., and Moser, E. I. 2005. Microstructure of a spatial map in the entorhinal cortex. Nature 436:801–6. doi: 10.1038/nature03721
[2] ↑ Moser, E. I., Kropff, E., and Moser, M. B. 2008. Place cells, grid cells, and the brain’s spatial representation system. Annu. Rev. Neurosci. 31:69–89. doi: 10.1146/annurev.neuro.31.061307.090723
[3] ↑ Jun, J. J., Steinmetz, N. A., Siegle, J. H., Denman, D. J., Bauza, M., Barbarits, B., et al. 2017. Fully integrated silicon probes for high-density recording of neural activity. Nature 551:232–6. doi: 10.1038/nature24636
[4] ↑ Steinmetz, N. A., Aydin, C., Lebedeva, A., Okun, M., Pachitariu, M., Bauza, M., et al. 2021. Neuropixels 2.0: A miniaturized high-density probe for stable, long-term brain recordings. Science 372(6539):eabf4588. doi: 10.1126/science.abf4588
[5] ↑ Zong, W., Wu, R., Li, M., Hu, Y., Li, Y., Li, J., et al. 2017. Fast high-resolution for brain imaging in freely behaving mice. Nat. Methods 14:713–9. doi: 10.1038/nmeth.4305
[6] ↑ Zong, W., Obenhaus, H. A., Skytøen, E. R., Eneqvist, H., de Jong, N. L., Vale, R., et al. 2022. Large-scale two-photon calcium imaging in freely moving mice. Cell 185:1240–56. doi: 10.1016/j.cell.2022.02.017
[7] ↑ Gardner, R. J., Hermansen, E., Pachitariu, M., Burak, Y., Baas, N. A., Dunn, B. A., et al. 2022. Toroidal topology of population activity in grid cells. Nature 602:123–8. doi: 10.1038/s41586-021-04268-7
[8] ↑ Moser, E. I., Roudi, Y., Witter, M. P., Kentros, C., Bonhoeffer, T., and Moser, M. B. 2014. Grid cells and cortical representation. Nat. Rev. Neurosci. 15:466–81. doi: 10.1038/nrn3766