Abstract
If you ever did the egg drop challenge, you know it is hard to build something that can protect a fragile egg from crashing into the ground and breaking. Engineers are building soft robots called tensegrity robots, which are designed to survive harsh crashes. The word tensegrity comes from “tension” and “integrity”. It means the robot is made of stiff bars held together with stretchy cables. This flexible structure helps a tensegrity robot absorb the impact from crashes. Someday, these robots might be used to explore dangerous places like deep caves or other planets. These robots could fall off cliffs or into craters. Right now, engineers are making tensegrity robots better and easier to control. In this article, we will explain how tensegrity robots work. We will discuss their advantages, their disadvantages, and what they can be used for.
Tensegrity Robots are Inspired by Art
Seventy years ago, an artist named Kenneth Snelson started to build the interesting sculptures you see in Figure 1 [1]. These sculptures have stiff bars tied together by tight cables. The cables make it look like the bars are floating. Snelson’s teacher was an architect named Buckminster Fuller. Fuller called these floating sculptures “tensegrity”. This word combines tension and integrity. Tension means the cables are tight. Integrity means the sculpture stays together. Fuller and other architects noticed that tensegrity sculptures are useful. They can be used to build structures like a roof or a bridge. A tensegrity is mostly empty space. Structures built with a tensegrity can be lightweight and even cost less money.
![Figure 1 - Two tensegrity sculptures by Kenneth Snelson [1].](https://www.frontiersin.org/files/Articles/1452937/frym-12-1452937-HTML-r2/image_m/figure-1.jpg)
- Figure 1 - Two tensegrity sculptures by Kenneth Snelson [1].
- (A) X-piece from 1948. (B) Easy landing in Baltimore from 1977.
Many years later, engineers started to build tensegrity robots (Figure 2) [3]. Tensegrity robots do not look like normal robots. Instead, they are made of stiff bars and tight cables, just like tensegrities. These robots have the same advantages as tensegrities—they are lightweight, flexible, and mostly empty space. You can even fold up tensegrity robots so that they are easy to send to faraway places like other planets.
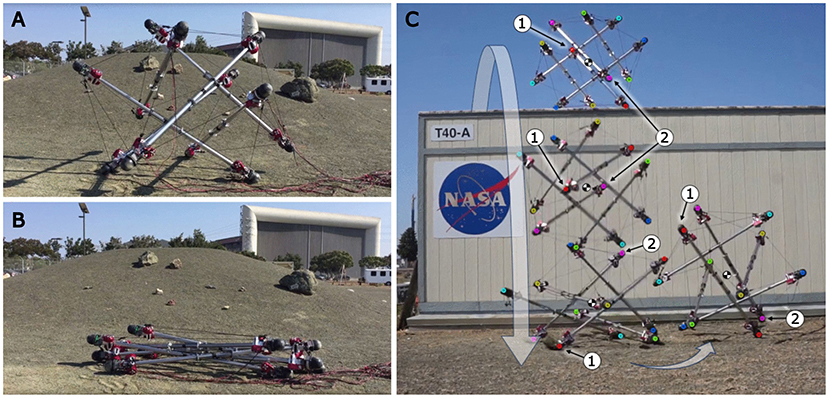
- Figure 2 - (A) NASA’s SUPERball tensegrity robot can roll on dirt.
- (B) It can pack itself flat. (C) It can also survive a fall from a roof. The engineers at NASA built SUPERball to explore other planets. Reprinted with permission from “Design of SUPERball v2, a compliant tensegrity robot for absorbing large impacts” [2].
There is one more big advantage to tensegrity robots: they can survive falling off a cliff! When a tensegrity robot crashes into the ground, its shape will deform to absorb the impact. You can understand how this happens if you have ever jumped on a trampoline. It does not hurt to fall on a trampoline because the trampoline is flexible and deforms when you land on it—unlike falling on cement! Tensegrity robots are also flexible like trampolines, so they absorb the energy from impacts. Engineers at NASA are excited to build tensegrity robots for this reason [2]. They want to make tensegrity robots that can crash land on other planets, or that can roll into craters to explore the bottom. Watch a video of NASA’s tensegrity robot here [2]. Future tensegrity robots will have to protect the scientific instruments they carry. That is even harder than the egg drop challenge from school! After it survives the drop, the tensegrity robot must explore its surroundings. But how can we build tensegrity robots and get them to move?
How Can We Make Tensegrity Robots Move?
If you stand on your tiptoes and lean forward, you will fall over. You might be surprised to learn that is how tensegrity robots move around! When you are standing up normally, your center of gravity is directly above your feet. Even if you lean forward a little bit, your center of gravity will still be above your feet, so you will not fall. When you stand on your tiptoes, the area your feet support is smaller, so now leaning forward even just a little can make you fall. Instead of feet, tensegrity robots have a structure called a polygon of stability as their bottom face. When a tensegrity robot shrinks its polygon of stability, it is like standing on tiptoes. Then, the robot can deform its shape and fall over, or roll over, by pushing its center of gravity outside its polygon of stability. The tensegrity robot can keep rolling by falling over again onto the next face.
Other tensegrity robots use different strategies for locomotion. In addition to rolling, some tensegrity robots crawl, jump, vibrate, and even swim. Most tensegrity robots move by using electric motors to make their cables longer or shorter. Other tensegrity robots use inflatable actuators to get their bodies to deform. Watch this video to see a crawling tensegrity robot with inflatable actuators [4]. There is a lot of variety when it comes to tensegrity robots, which makes them cool and exciting to study. But it can be difficult to design a new tensegrity robot because there are so many choices to make.
Simulations Help Us Design and Control Tensegrity Robots
Building robots can cost a lot of money. Sometimes we do not know what type of robot to build for a task. Even when we do, we might not know how to get it to move. It is easy to understand the locomotion of a car—spin the wheels to move forward and turn the front wheels to turn. Unlike a car, tensegrity locomotion is not obvious. Which cables should the robot pull to move forward or turn? Engineers solve these problems by making simulations on a computer. A simulation is like a video game. In a video game, you can control a character, making it run and jump. The game calculates where you land using physics and math. Simulations do the same thing, but for robots. Simulations predict what a robot will do when we give it some controls, which helps us figure out the best controls. The simulation can also tell us how good a robot will be before we build it.
We can make simulations with lots of different tensegrity robots. These robots can have different numbers of bars, different shapes, and different sizes. Then, we can compare their speeds or another property to choose which robot is the best one. We can even use the simulation to find out the best strategy for locomotion. Just like you can run, walk, crawl, and swim, tensegrity robots have different ways they can move around. The best way to move depends on the surroundings. Simulations tell us if a certain tensegrity robot can roll or crawl, and they also tell us which cables it needs to pull on to move a certain way. In this video, we use a simulation to learn how to control a 3-bar tensegrity robot [5].
It is hard to make simulations of tensegrity robots because these robots are flexible, which makes it more complicated to predict what they will do. Also, when a tensegrity robot pulls on one cable to make it shorter, that will affect all the other cables in the tensegrity. Even with these challenges, researchers keep making better simulations for tensegrity robots [5]. No matter how hard we try, we will never simulate a robot perfectly. This problem is called the sim2real gap. There are lots of things in the real world that are hard to measure precisely. Even if we could, the real world has some randomness that is impossible to predict. Simulations are useful for figuring out how to design and control tensegrity robots. Still, we always must build the real robot and test it to check the simulation. Then, we can be happy if our simulation is accurate!
Tensegrity robots in the future
When Kenneth Snelson was making art sculptures 70 years ago, he did not know that his work might lead to robots that explore other planets. Today, engineers keep designing new and better tensegrity robots. One day, tensegrity robots might search underground caves [6] or the bottoms of craters (Figure 3). They might do other things we have not even imagined. No one thought art would become a robot one day! There are still problems to solve, though. Tensegrity robots can be difficult to design, simulate, and control. Tensegrity robots are also not as reliable as robots with wheels or even legs. More research is needed for tensegrity robots to reach their full potential. Maybe you will be the one to solve these problems!
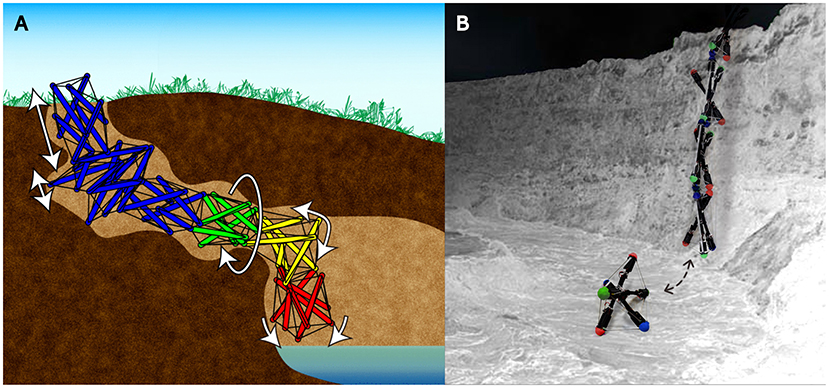
- Figure 3 - These illustrations show the future of tensegrity robots.
- (A) Engineers in Japan are building tensegrity robots to explore underground caves. Reprinted with permission from “large torsion thin artificial muscles tensegrity structure for twist manipulation” [6]. (B) We think tensegrity robots will also explore craters on other planets.
Glossary
Tension: ↑ A pulling force that makes things tight when you pull on them, like strings, ropes, cords, and cables.
Integrity: ↑ A structure with many parts that are attached and stay together.
Egg Drop Challenge: ↑ An activity that kids do in some schools and summer camps. Design something to protect an egg when it gets dropped from very high.
Center of Gravity: ↑ The point on your body where Earth’s gravity pulls on you. In physics calculations, this point can be thought of as where all your weight is located.
Locomotion: ↑ A robot or another machine moving itself from one place to another.
Actuator: ↑ The part of the robot that makes it move, like a motor.
Simulation: ↑ A computer program that uses physics or data to predict how a robot will move.
Sim2real Gap: ↑ The small (or large) differences between simulations and the real world. No simulation can ever be perfect, so there will always be a gap between simulation and reality.
Conflict of Interest
The authors declare that the research was conducted in the absence of any commercial or financial relationships that could be construed as a potential conflict of interest
Acknowledgments
This material was based upon work supported by the National Science Foundation under grant no. IIS-1955225.
References
[1] ↑ Micheletti, A., and Podio-Guidugli, P. 2022. Seventy years of tensegrities (and counting). Arch. Appl. Mechan. 92:2525–48. doi: 10.1007/s00419-022-02192-4
[2] ↑ Vespignani, M., Friesen, J. M., SunSpiral, V., and Bruce, J. 2018. “Design of superball v2, a compliant tensegrity robot for absorbing large impacts”, in 2018 IEEE/RSJ International Conference on Intelligent Robots and Systems (IROS) (Madrid: IEEE), 2865–71.
[3] ↑ Shah, D. S., Booth, J. W., Baines, R. L., Wang, K., Vespignani, M., Bekris, K., et al. 2022. Tensegrity robotics. Soft Robot. 9:639–56. doi: 10.1089/soro.2020.0170
[4] ↑ Kobayashi, R., Nabae, H., Endo, G., and Suzumori, K. 2022. Soft tensegrity robot driven by thin artificial muscles for the exploration of unknown spatial configurations. IEEE Robot. Automat. Lett. 7:5349–56. doi: 10.1109/LRA.2022.3153700
[5] ↑ Wang, K., Johnson, W.R., Lu, S., Huang, X., Booth, J., Kramer-Bottiglio, R., et al. 2023. “Real2sim2real transfer for control of cable-driven robots via a differentiable physics engine”, in 2023 IEEE/RSJ International Conference on Intelligent Robots and Systems (IROS) (Detroit: IEEE), 2534–41.
[6] ↑ Kobayashi, R., Nabae, H. and Suzumori, K., 2023. Large torsion thin artificial muscles tensegrity structure for twist manipulation. IEEE Robot. Automat. Lett. 8:1207–14. doi: 10.1109/LRA.2023.3236889