Abstract
In the field of particle physics, we try to understand what our universe is made of. We study the basic properties of matter, describe the elementary particles that compose matter, and try to understand how the various particles that build our world work together. In this article, we will dive into the heart of atoms—the building blocks of matter—and try to answer some intriguing, basic questions about the universe such as: “What are protons and neutrons, the particles that make up the nucleus, made of?” and, “Can those components be divided into even smaller particles?”
Professor David Gross was awarded the Nobel Prize in Physics in 2004, jointly with Prof. Hugh David Politzer and Prof. Frank Wilczek, for the discovery of asymptotic freedom in the theory of the strong interaction.
Theoretical Physics—My Middle School Sweetheart
I was curious about science from a young age. When I was in middle school, I was enormously interested in reading popular science books, such as One Two Three…Infinity by George Gamow, which explores concepts in mathematics and physics. For my 13th birthday, I got a very special book signed by no other than Albert Einstein himself. It was given to me by a relative of Einstein’s collaborator, Leopold Infeld, who co-authored the book. That was when I fell in love with theoretical physics. I was fascinated by the idea of using math and my own mind to understand the universe. From then on, I knew I wanted to be a theoretical physicist, and indeed I became one—and still am. My love for theoretical physics has matured with the years, but it is basically the same love I felt as a teen. I am still excited to understand the basic puzzles of our universe, one of them being: What is the universe made of?
Studying What Matters With Particle Physics
What exactly is matter? What is it composed of? These are the kinds of questions we try to address in particle physics. Nowadays, it is common knowledge that all matter—from the stars to our own bodies—is made of atoms, which are themselves made of protons, neutrons, and electrons. Up until the early 1900s, the structure of the atom was not understood. Between 1908 and 1913, a physicist from New Zealand named Ernest Rutherford and his students performed a series of experiments to explore the structure of the atom [1]. They took tiny particles called alpha particles and smashed them into the atoms in a sheet of gold foil (To read more about Ernest Rutherford and this experiment, see here and here). Some of the alpha particles passed through the foil uninterrupted, while others seemed to have bumped into something stiff and scattered off in various directions (Figure 1A). The results of these famous experiments revealed that most of the volume of the atom is empty space, while most of its mass and all of its positive charge are concentrated in a very small volume in its center, called the nucleus. This was a great discovery, and it marked the beginning of particle physics.
A few years later, Rutherford discovered that the positive charge of the atom is created by particles called protons, and that the number of protons in an atom is equal to the number of electrons circling the nucleus [2]. Then, it took more than 10 years to make the next step toward understanding the structure of the nucleus. In 1932, a renowned physicist named James Chadwick discovered that the nucleus contains protons and neutrons [3]. Chadwick’s discovery completed our basic understanding of the classic “solar system” model of the atom, in which electrons orbit around the nucleus (Figure 1B). But what are protons and neutrons made of?
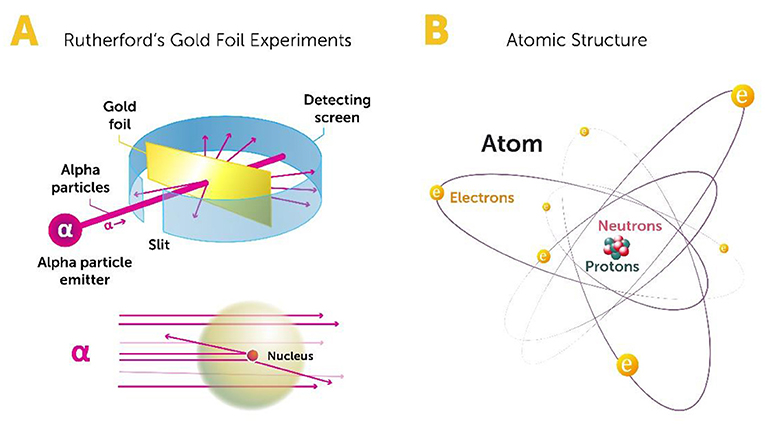
- Figure 1 - Early studies of the atom’s structure.
- (A) Rutherford’s gold foil experiment in 1919 revealed that the atom has a solid nucleus at its center. When alpha particles were used to probe the atoms in a sheet of gold foil, some of them passed right through while others bounced off something that changed their path. This obstacle turned out to be the nucleus (Image adapted from: https://flexbooks.ck12.org/cbook/ck-12-chemistry-flexbook-2.0/section/4.14/primary/lesson/rutherfords-atomic-model-chem/). (B) Studies in the 1930s revealed that the atom has a solar system-like structure, in which electrons orbit around the nucleus.
Speeding Up Our Understanding With Accelerators
Since Rutherford’s pioneering gold foil experiment, particle physicists have done many similar experiments, scattering one particle, called a probing particle, off a different particle, called a target particle, to learn about the properties of the target particle. This process is called probing. We measure the scattering of the probing particle (for example, an electron) off the target particle (for example, a proton), when the probing particle is sent at various angles and with various amounts of energy. This information allows us to infer the structure of the target particle. To understand probing, we can use the following analogy: Imagine a room full of people. You want to know where the people are, but you cannot get into the room. What you can do is throw balls into the room. So, you throw one ball in and nothing happens. Then you throw another ball, at a slightly different angle, and you hear “Ouch!”, so you know there is a person in that direction. If you throw many balls in many different directions and at various speeds, you can eventually get a sense of how the people are organized in the room. The same principle applies when we scatter one particle off another—we learn about the structure of the target particle from the feedback we get from the scattering of probing particles.
To probe the structure of the nucleus, Rutherford scattered alpha particles off it, which he could do in his lab. If we want to zoom even deeper into matter, probing the structure of subatomic particles like protons and neutrons, or if we want to discover new subatomic particles, we must use probing particles with much higher energies than Rutherford used. The reason is that subatomic particles are held together by strong forces, so we must use great energies to break them apart so we can study their structures. To do this, we use accelerators—devices that accelerate the probing particles to very high speeds.
At the beginning of my scientific career in the 1960s and 1970s, new accelerators were being built and used. Two accelerators that influenced my career were the Bevatron at the University of California, Berkeley, where I was a graduate student, and the linear accelerator at the Stanford Linear Accelerator Center (SLAC) at Stanford University (Figure 2). Compared to the strongest accelerators we have today (notably the Large Hadron Collider at CERN), the Bevatron and the linear accelerator could not accelerate the probing particles to very high velocities. In fact, they managed to produce energies of about six giga electron volts (GeV; one electron volt equals the energy that one electron has when it is accelerated by a voltage of one volt), which is about a thousand times less than the energies of today’s accelerators. Yet, those accelerators were strong enough to allow scientists to discover new particles on a weekly basis. This was an extremely exciting time in particle physics, and I knew I wanted to be where the great action was. Even though experiments were flourishing, we had very little theoretical understanding of the structures of subatomic particles and the interactions that govern their behavior. I chose to focus on a very basic problem: what is the proton made of?
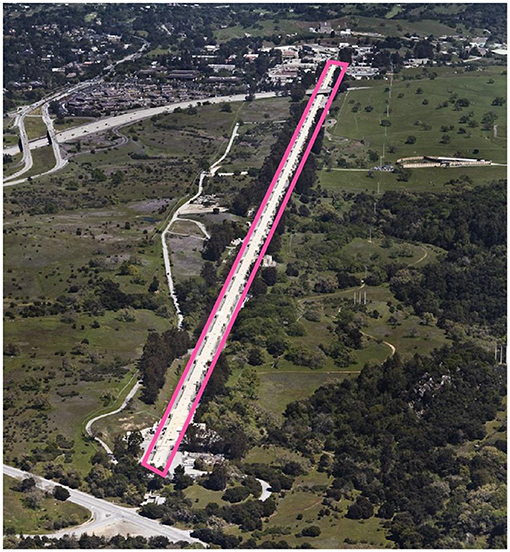
- Figure 2 - The SLAC linear accelerator at Stanford University.
- The SLAC accelerator (highlighted in pink) was constructed in 1966. It is 2 miles (3.2 kilometers) long and is capable of accelerating electrons to energies of 50 giga electron volts (Image source: https://upload.wikimedia.org/wikipedia/commons/e/ef/SLAC_National_Accelerator_Laboratory_Aerial.jpg).
The Structure of Protons
At SLAC’s linear accelerator, electrons, which are simple point particles (meaning particles concentrated into an extremely small volume) were used to probe protons to study their structure. At that time, no one knew what protons were made of. One hypothesis was that the proton, like the electron, was a point particle, and that it was not composed of any smaller parts. Another hypothesis was that the proton was made of an evenly distributed, unknown material. Surprisingly, the results from the SLAC accelerator did not fit either hypothesis, but seemed to indicate that the proton was made out of other point particles. However, no one had ever seen the point particles that supposedly composed the proton, and no matter how hard we hit the proton with the probing particles, those point particles did not come out (In contrast, when we smash atoms together, their electrons fly off.). Additionally, probing results seemed to indicate that the point particles in the proton were bouncing around as if no force was acting between them. That was very strange because we knew that the point particles were bound tightly within protons—but we could not explain what was keeping them bound there.
In my search for an explanation of these surprising findings, I was assisted by two previous theories. One theory was proposed by physicists Murray Gell-Mann and George Zweig in the early 1960s [4, 5]. Gell-Mann and Zweig realized that the data emerging from the measurements of strongly interacting subatomic particles could be explained mathematically, by assuming that they were made of three kinds of more basic particles that Gell-Mann called quarks (Figure 3A). At first, this assumption was thought to be only a mathematical “trick” that had nothing to do with reality. But, as time went on, some of the predictions of this model turned out to be pretty accurate, and together with new results from accelerator experiments the idea of quarks seemed to be worth exploring in greater detail. The other theory I used was generalizations of Maxwell’s theory of electromagnetism. Maxwell’s theory explains the force of electricity and magnetism based on the existence of a single type of charge, called the electrical charge.
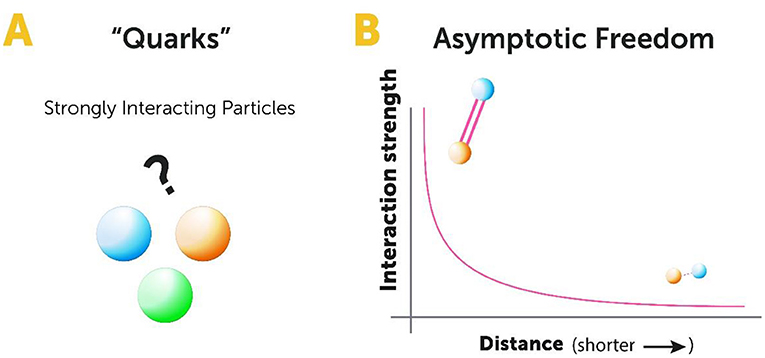
- Figure 3 - Studying subatomic particles.
- (A) In the 1960s, physicists Murray Gell-Mann and George Zweig suggested that subatomic particles, like protons and neutrons, are made of basic particles called quarks. Quarks are called strongly interacting particles because they are bound by the strong force. (B) In the 1970s, my colleagues and I found a mathematical explanation of the behavior of quarks—a phenomenon called asymptotic freedom. Asymptotic freedom describes how the interaction between quarks becomes weaker as they come closer together and stronger as they move further apart. This explains why quarks stay “locked” within protons, which is called confinement.
Generalizations can be made from Maxwell’s theory, such that other kinds of charges can be used to explain other natural forces. In physics, there are four basic forces: the electromagnetic force, which is responsible for electricity and magnetism; the weak force, which is responsible for radioactivity (to learn more about radioactivity, see here); the strong force, which binds protons and neutrons in the nuclei of atoms; and gravity, which pulls massive objects toward one another. I was trying to explain the strong force holding protons and neutrons together.
After doing some complicated math based on these two theories, I developed a new theory called quantum chromodynamics (QCD). QCD explains the properties of quarks and the forces that act on them. In QCD, there are three types of charges (opposed to only one type—electrical—in classical electromagnetism) and eight types of forces that act between these charges. One of QCD’s greatest achievements is its ability to explain the surprising finding that quarks behave like free particles inside the proton. My colleagues and I found a mathematical explanation for why the strong force that acts between quarks becomes weaker and weaker as the quarks get closer together—a phenomenon called asymptotic freedom [6, 7] (Figure 3B). The discovery of asymptotic freedom led to a great advancement in particle physics, for which I was awarded the Nobel Prize in Physics in 2014, jointly with Hugh David Politzer and Frank Wilczek. Asymptotic freedom was a surprising discovery because other forces in nature get weaker as the particles move further apart. Asymptotic freedom explains why we do not see free quarks—the further we try to separate them, the stronger the force pulling them together becomes. This means that quarks stay “locked” inside protons—a phenomenon called confinement.
Quirky Quarks: Surprising Building Blocks of Matter
We currently believe that quarks are point particles that make up protons, neutrons, and a whole class of strongly interacting particles called hadrons. As we have seen, quarks are confined within the particles they constitute, and they move like small balls bouncing around inside a larger ball. Quarks have three types of charges: electric charge, flavor, and color (not the same “flavor” and “color” in the traditional sense that we detect with the tongue or eyes). In terms of electric charge, quarks can have a charge that is some fraction of the charge of an electron or proton: either ( of the negative charge carried by an electron) or ( of the positive charge carried by a proton). Quarks come in six flavors, which we call up, down, strange, charm, top, and bottom (Figure 4A). Quark flavors are related to the weak force responsible for radioactivity. Protons are made of two “up” quarks and one “down” quark; neutrons are made of two “down” quarks and one “up” quark (Figure 4B). In addition to electric charge and flavor, quarks can come in one of three colors: red, white, and blue. Colors are the source of the strong force that binds quarks together, and this is also the source of the name chromodynamics—“chromos” means “color” in Greek.
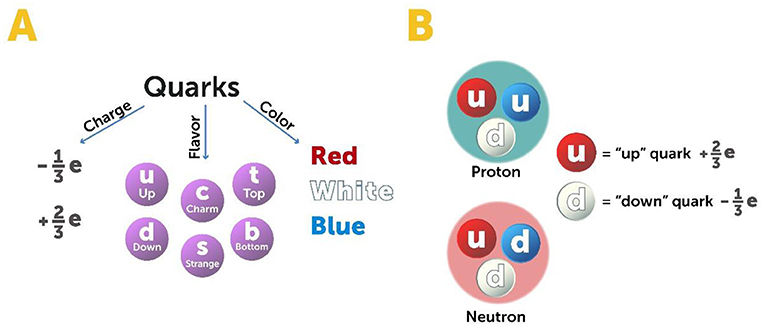
- Figure 4 - Quarks.
- (A) Quarks come in various electric charges, flavors, and colors. Their charge is some fraction of the charge of an electron or proton. They come in one of three colors, which are the source of the strong force that binds them together, and in one of six flavors which are related to the weak force. (B) Protons are made of two “up” quarks and one “down” quark, while neutrons are made of two “down” quarks and one “up” quark.
At this stage, you might be wondering whether quarks themselves are made up of even smaller particles. In the history of physics, we have progressively found smaller and smaller particles that make up matter: atoms are made of electrons and nuclei, nuclei are made of protons and neutrons, protons and neutrons are made of quarks…maybe quarks are made up of something else? So far, there is no experimental evidence to indicate that quarks are made of sub-quarks. To get definite experimental proof that quarks are truly indivisible, our accelerators will need extraordinarily high energies—of the order of 100 trillion trillion eV, which is about a trillion (or 1012) times higher than the energies we currently use. This is very difficult and costly, and the path to getting there is still unclear. On the theoretical side, we can extrapolate our current knowledge of the basic forces of nature to very short distances. When we do so, we find that gravity should play an important role at these short distances, equivalent to that of the strong force. With our current models, we do not understand this interplay between gravity and the strong force, so something unexpected might be occurring at very short distances. Experiments indicate that something is still missing in our theory, and study of quarks might help us overcome some of the current gaps in our understanding of the basic building blocks of the universe.
String Theory: An Alternative Perspective on Matter
String theory, which many scientists (myself included) have been working on for several decades, is another interesting theory that could offer us a new way of understand the universe. String theory is extremely complex mathematically, and its details exceed what can be described in this article. But, in principle, string theory is an attempt to understand how all the elementary particles, including quarks, could be made of one type of object, called a string. This string can vibrate in many different ways, and each vibrational pattern corresponds to a specific elementary particle. For example, if the string vibrates in one specific pattern we get a quark, if it vibrates in a different pattern we get an electron, and so on (Figure 5). If string theory is correct, then quarks and other elementary particles do not contain additional sub-particles but are instead made of vibrating strings. Testing string theory experimentally will require energies much more powerful than we currently have—so it might take a while, but I think this is an exciting development to look forward to.
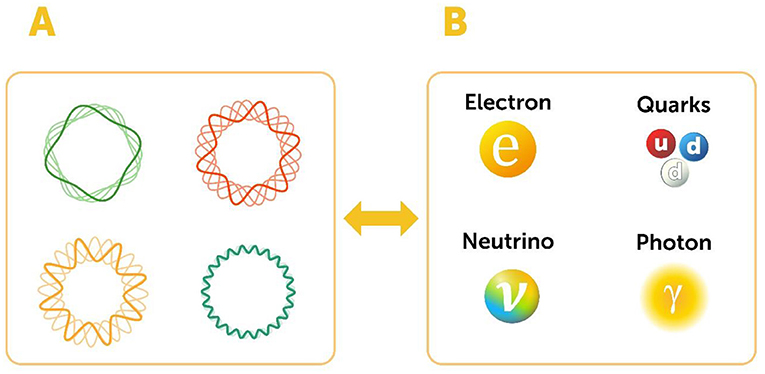
- Figure 5 - String theory.
- (A) String theory suggests that all elementary particles are composed of an object called a string. (B) When this string vibrates in different ways, specific particles are produced. We do not currently have the technology to test whether string theory is true, but hopefully we will be able to do so in the future.
Recommendations For Young Minds
In physics, as in all of science, it is important for scientists to be creative. Unfortunately, we do not know how to teach people to be creative, but we can serve as examples of creativity and be inspiring mentors for our students. The best thing students can do is observe how creative and successful people work and learn their “tricks.” I see my students as colleagues, and I like to work with them as equal contributors. That might be challenging for some students, but others flourish in that type of relationship. Prof. Frank Wilczek, my first official student, won the Nobel prize with me for our shared work on asymptotic freedom—he is a good example of a student who flourished by being treated like an equal.
To become good scientists, it is also important to start doing research as soon as possible. Research is very different from taking classes and studying. In physics class, problem sets are the best method we have to prepare students to do research. We give students lots of problems, and they must solve those problems and usually gain insights while doing so. But even the best problems students get in class are made up. In contrast, research is based on real, unresolved problems that no one knows in advance how to solve. The biggest challenge in research is to come up with the right questions. Before you even tackle a scientific question, you must first make sure that the question is a good one (Figure 6). Asking good questions is also a skill we cannot teach directly—we can only supply examples and model productive ways of thinking. Once you manage to ask a good question, you might not be able to answer it right away, but at least you can make progress toward an answer.
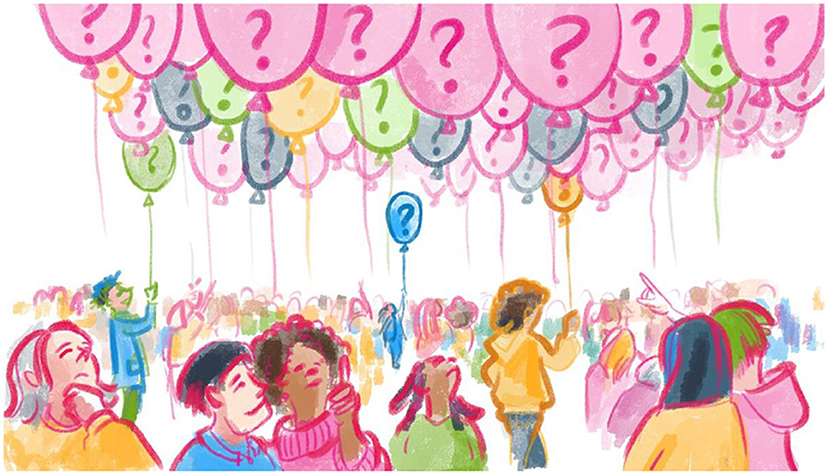
- Figure 6 - Recommendations for Young Minds.
- One of the most important skills needed for doing good research is coming up with good research questions.
In my view, there are many reasons why being a scientist can lead to a happy life. For one, society generally respects the talent that it takes to be a scientist, because science is so important to our lives. Therefore, science allows you to both be supported and respected by society and to make a living, which is a great pleasure. It feels good to be in a field where your work is your play, your passion, and your interest. Another advantage of being a scientist is that you belong to a world-wide, international community of people who share the same passions and interests. You can go anywhere in the world and find people who are interested in the same topics you are, and have interesting discussions with them. Science is a kind of family that you choose to join.
I think that people should do what they love doing. It does not have to be science—it could be anything. If you can spend your life doing what you really enjoy, you are very lucky. This is my best advice about how to decide what to do with your life—find out what you enjoy and what you are good at. Then, be ambitious—try hard and be willing to fail. If you have chosen a path that you enjoy, the joy that you receive is worth the chance of failure.
Glossary
Theoretical Physics: ↑ A branch of physics that uses mathematical equations to build models that help to describe the world.
Particle Physics: ↑ A branch of physics that studies the building blocks of matter.
Subatomic Particles: ↑ Particles that make up atoms and are therefore smaller than the size of an atom.
Accelerators: ↑ Devices that accelerate particles to very high speeds and collide them with target particles, to study the structure of the target particles.
Radioactivity: ↑ A process by which particles and/or energy are emitted from an unstable atom.
Strong Force: ↑ The force that binds protons and neutrons in the nuclei of atoms.
Asymptotic Freedom: ↑ A phenomena whereby quarks act like free particles when they are short distances apart, but oppose being separated from one another as the distance increases.
Confinement: ↑ The property of quarks to stay “locked” inside protons (or other particles), even when great forces are applied to separate them.
Hadrons: ↑ Particles that are built from quarks and interact via the strong force.
String Theory: ↑ A theory of physics that describes the basic particles of nature in terms of a string that vibrates in different ways.
Conflict of Interest
The author declares that the research was conducted in the absence of any commercial or financial relationships that could be construed as a potential conflict of interest.
Acknowledgments
I wish to thank Noa Segev for conducting the interview which served as the basis for this paper, and for co-authoring the paper, and Alex Bernstein for providing the figures.
Additional Materials
References
[1] ↑ Rutherford, E. 1911. LXXIX. The scattering of α and β particles by matter and the structure of the atom. Lond. Edinburgh Dublin Philos. Magaz. J. Sci. 21:669–88. doi: 10.1080/14786440508637080
[2] ↑ Rutherford, E. 2010. Collision of α particles with light atoms. IV. An anomalous effect in nitrogen. Philos. Magaz. 90:31–7. doi: 10.1017/CBO9780511707179.010
[3] ↑ Chadwick, J. 1932. The existence of a neutron. Proc. R. Soc. Lond. Ser. A, Contain. Papers Math. Phys. Charact. 136:692–708. doi: 10.1098/rspa.1932.0112
[4] ↑ Gell-Mann, M. 1961. The Eightfold Way: A Theory of Strong Interaction Symmetry. Synchrotron Laboratory Report CTSL-20. California Institute of Technology. doi: 10.2172/4008239
[5] ↑ Zweig, G. 1964. An SU3 Model for Strong Interaction Symmetry and Its Breaking (No. CERN-TH-412). CM-P00042884.
[6] ↑ Gross, D. J., and Wilczek, F. 1973. Ultraviolet behavior of non-abelian gauge theories. Phys. Rev. Lett. 30:1343. doi: 10.1103/PhysRevLett.30.1343
[7] ↑ Gross, D. J., and Wilczek, F. 1973. Asymptotically free gauge theories. I. Phys. Rev. D 8:3633. doi: 10.1103/PhysRevD.8.3633