Abstract
Imagine you could choose a new set of eyes that would help you see things you have never been able to see before. Maybe you would choose Superman’s X-ray vision, or maybe you would prefer to zoom into tiny things and see the wonders of the microscopic world. Science has recently gained a new set of eyes—a new way to look into the mysteries of the universe—using gravitational waves, which are waves produced by gravity itself. In this article, I will take you on a journey that begins with an explanation of gravity—from the classical perspective of Isaac Newton to the modern and more complex view of Albert Einstein. I will then explain how movements of massive objects create gravitational waves, which are ripples in space and time, and how they might be used to explain some mysteries of the universe, and even help us to understand the origins of our planet Earth.
Professor Barish won, jointly with Profs. Rainer Weiss and Kip Thorne, the Nobel Prize in Physics in 2017 for decisive contributions to the LIGO detector and the observation of gravitational waves.
Gravity—from Newton to Einstein
In 1687, the great English mathematician and physicist Sir Isaac Newton published his famous book, Principia [1], in which he presented his theory of gravity—the first “universal” theory in science. Newton’s theory proved that the gravitational force between two objects is proportional to the product of their masses and inversely proportional to the square of how far apart they are. This sounds complicated, but it means that the more mass the objects have, and the closer they are to each other, the stronger the gravitational force they have on each other. While this is true, it turned out that Newton’s wonderful theory has a few limitations.
First, have you ever wondered why, when an apple falls from a tree, it falls down and not up? When you jump, why do you come back down to Earth rather than flying upwards? Newton’s theory does not actually answer these simple questions. It only tells us the amount of gravitational force the two objects exert on each other, like the force between the apple and the Earth or between you and the Earth. Newton’s theory does not consider the direction of the force between objects (toward each other or away from each other) nor does it explain where gravity comes from in the first palace (Figure 1).
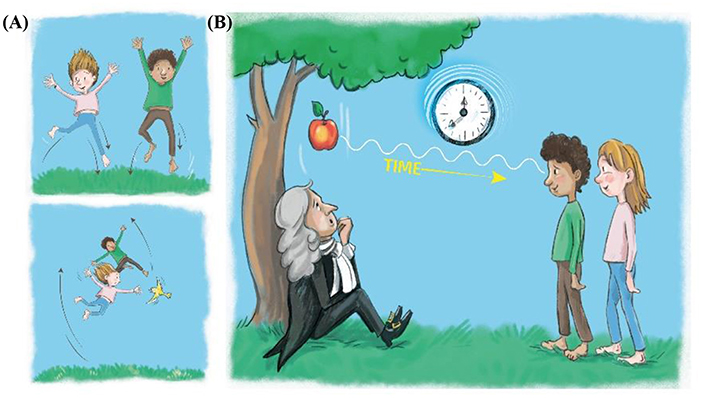
- Figure 1 - Isaac Newton’s successful theory of gravity has several limitations.
- (A) Have you ever wondered why you fall back to Earth when you jump up, instead of flying into the sky? What attracts you back to Earth? Newton’s theory could not answer this question. (B) When an apple falls from a tree, it takes time for an observer to know that it happened, because the information travels at the speed of light. Newton’s theory assumes that the observer sees the apple falling instantaneously, at exactly the same moment that it falls. Both limitations were solved by Einstein’s theory of gravity.
The second difficulty with Newton’s theory is a little harder to grasp. Imagine that the Sun suddenly disappeared. If it disappeared right now, it would take about 8 min before we could see that it was no longer there, because it takes 8 min for light to reach us from the Sun. The same is true for everything else that happens in the universe—it takes time for the information to travel from the event to the observer. So, when an apple falls from a tree, it should take some time (even if only a tiny fraction of a second) for the observer to know what actually happened (Figure 1). Newton’s theory does not take this time interval into account so, according to his theory, the observer sees the apple falling at exactly the same moment that it actually falls. We know that this is not the case in reality; therefore, we can conclude that something is missing in Newton’s theory.
How can we solve these two puzzles posed by Newton’s theory? Luckily, more than 200 years after Newton, the beloved physicist Albert Einstein produced a solution. In 1915, Einstein published a new theory of gravity called the Theory of General Relativity [2]. Einstein’s theory has a completely different way of looking at gravity, and it helps us to understand things that Newton’s theory was unable to explain. This does not mean that Newton’s theory was wrong or unhelpful—it just means that it was incomplete, and that the newer theory helps us understand things in a deeper way. Einstein’s theory says that, around any massive object, space and time are affected and get distorted or curved, and this creates a pull toward that object.
Here is a simple way to understand Einstein’s idea of gravity. Imagine placing a marble on a flat trampoline. The marble stays still and does not move (Figure 2A). However, if you put a large bowling ball at the center of the trampoline, which makes the trampoline curve, the marble will fall toward the center of the trampoline (Figure 2B). The presence of the heavy bowling ball distorted the space occupied by the trampoline in a way that made the marble move toward the bowling ball, as if attracted by it. That is basically what happens in Einstein’s theory of general relativity. The presence of any mass distorts the space around it in a way that creates an attraction between masses. This picture of gravity answers the question that Newton could not answer: why (and how) does gravity create an attractive force, and why do you fall toward the Earth when you jump up? The second problem, which relates to time, was also solved by Einstein because his theory takes the speed of light into account. In the next section, we will see an interesting and important phenomenon called gravitational waves, which Einstein’s theory of gravity predicts.
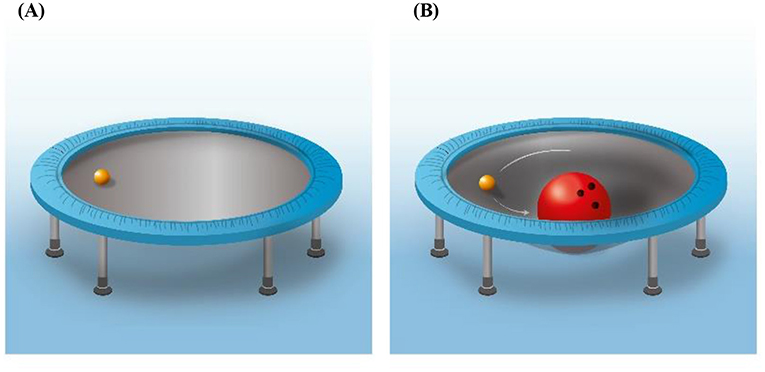
- Figure 2 - Gravity according to Albert Einstein.
- (A) When you put a marble on a flat trampoline, it stays in place. This represents the situation of space when there are no massive objects present. (B) When you put a heavy bowling ball in the middle of the trampoline, the trampoline becomes curved. If you now place the marble on the trampoline, it will move toward the center. This represents the gravity in Einstein’s model, where a massive object (like a star) curves space and time, and therefore attracts another object (like an apple, or yourself) toward it.
What are Gravitational Waves?
One of the predictions of Einstein’s theory of general relativity is that gravity should have waves—gravitational waves [3, 4]. A simple way to think about gravitational waves is to imagine yourself by a still pond…then you throw a rock in the pond. The rock makes a splash and drops to the bottom of the pond. Although the rock is now resting at the bottom of the pond, you can still see the effect it had on the surface of the water, where waves are moving from the center outwards (Figure 3A). This is also the way to visualize what happens with gravitational waves. What makes a gravitational wave is not a rock falling into a pond, but rather the motion or collision of massive objects in space (Figure 3B).
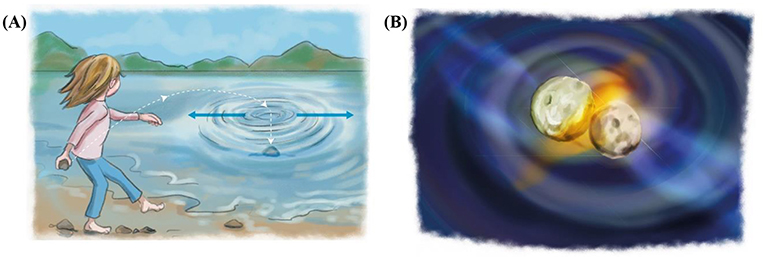
- Figure 3 - Gravitational waves.
- (A) When you throw a rock into a quiet pond, you can see ripples (waves) moving across the surface of the water, even when the rock is already standing still at the bottom of the pond. According to Einstein’s theory of general relativity, this is similar to the way gravitational waves are formed when massive objects collide. (B) Gravitational waves are created when two massive objects collide with each other. The waves continue to travel through space, even after the collision has happened.
Challenges and Successes of Detecting Gravitational Waves
After Einstein’s theory predicted the existence of gravitational waves, experimental physicists started trying to detect them. I myself have dedicated more than 20 years of my life developing methods to detect gravitational waves—and I am still doing so. It turns out that, when it comes to gravitational waves, we have both a big misfortune and a big fortune. The misfortune is that we cannot currently make gravitational waves in our laboratories because they are just too weak for us to detect with the techniques we have available. This is a misfortune because good experiments are ones where we understand all that is going on, and that is accomplished much more easily in the laboratory.
On the other hand, we were blessed with a great fortune—nature itself creates gravitational waves that are much stronger than any we could make in the laboratory. This means that some astronomical events that create gravitational waves—two of which I will mention below—can potentially be detected with our present, state-of-the-art detectors. Though these events must be the most violent and energetic astronomical events in the universe for us to detect them, they still occur frequently enough to study. The most violent events in the universe are explosions and collisions of extremely heavy objects.
One excellent possible source of gravitational waves that we can detect is a type of explosion called a supernova. A supernova happens when a massive star gets old and collapses rapidly inward. The collapse creates a huge increase in temperature and pressure, which can enhance the nuclear fusion, when lighter nuclei in atoms are combined into heavier nuclei and release energy. This can trigger what is called a “runaway nuclear fusion,”1 which causes the star to explode with enormous energy creating, according to Einstein’s theory, strong gravitational waves.
When it comes to violent collisions in space, some of the most energetic are between massive objects such as black holes and neutron stars. Black holes are the most massive objects known in the universe, and they have such powerful gravitational attraction that they “swallow” anything that comes near them, even stars. Nothing can escape from inside black holes, not even light—hence their name. Neutron stars are the remains of supergiant stars that have collapsed and are extremely dense and consist mainly of neutral subatomic particle called neutrons.
In 2015, the first gravitational waves were discovered [5]. With my two colleagues, Rainer Weiss and Kip Thorne, I won the Nobel Prize in Physics for this discovery, just two years later, in 2017. Usually, it takes at least 20 years before scientists receive a Nobel Prize for their work, but the discovery of gravitational waves was of special significance, for reasons I will explain below. Since those first observations of gravitational waves from the collision of two black holes, we later detected other collisions that produced gravitational waves—one in 2017, between two neutron stars [6], and another in 2020, between a black hole and a neutron star [7].
Measuring Gravitational Waves
When we measure gravitational waves, we actually measure the distortions (ripples) that they create in space and time. When these distortions arrive at our detectors, they are incredibly small—much smaller even than the size of a single proton. To measure such small signals, our detectors must have greater accuracy than 1/1,000 the size of a proton! As you can imagine, this is extremely hard to achieve, and it requires the use of a very special technique called interferometry. I will not describe it here in detail, but interferometry uses the interactions between laser beams to spot very small contractions and expansions of space2. To make such sensitive measurements, we need to isolate our equipment so nothing can disturb our measurements—even a tiny movement could swamp the signal we are looking for. One source of disturbance is the movement of Earth itself, which shakes as it revolves on its axis (this shaking is too mild to be felt by humans, but it is detectable by sensitive instruments). This means that we need to float our measuring instrument so that it does not pick up the Earth’s movements.
It has been extremely challenging to build instruments for measuring gravitational waves. The instrument we use is called LIGO, which stands for Laser Interferometer Gravitational-Wave Observatory. LIGO is a few kilometers long (Figure 4). Building and operating it cost more than 1 billion dollars. Much of my work still involves developing the technologies that enable us to achieve greater sensitivity in the detection of gravitational waves, without unwanted movements ruining our measurements. Many people ask me if it is frustrating to work on the same problem for more than 20 years. My answer is absolutely not! I have had a great deal of fun solving problems along the way, and it is a great privilege to do something that no one has ever done before.
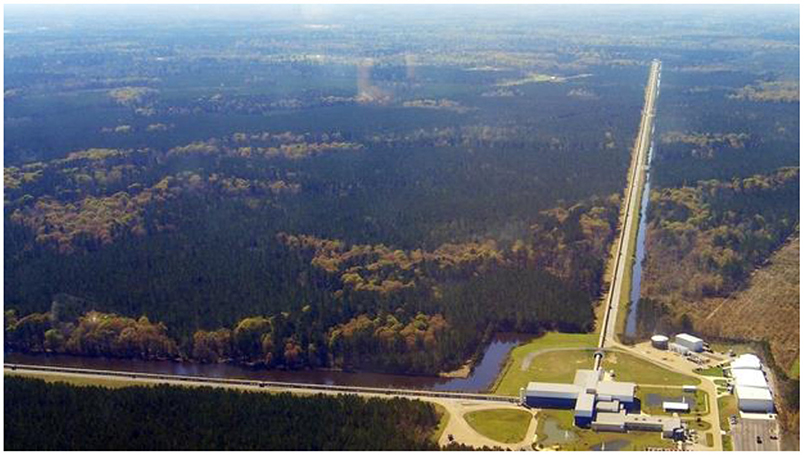
- Figure 4 - The LIGO Gravitational Waves Detector (Livingston, Louisiana, United States).
- A bird’s-eye view of one of the two gravitational wave detectors that detected the first-ever measured gravitational waves in 2015. Each LIGO detector consists of two arms, each 4 km (2.5 miles) long, made of 1.2-m-wide steel vacuum tubes arranged in an “L” shape, and covered by a 3-m wide, 3.7-m tall concrete shelter that protects the tubes from the environment. LIGO can detect gravitational waves coming from any direction, even from below (Photograph credit: Caltech/MIT/LIGO Lab).
Gravitational Waves: The New Window on the Universe
So, what is the importance of gravitational waves when trying to understand the universe? First, gravitational waves help us verify that Einstein’s theory of general relativity is indeed correct. Even though Einstein’s theory seems valid and very accurate, it is not the only theory that predicts gravitational waves. To confirm that Einstein’s theory is correct and can explain what gravity is and how it functions, we need to measure details of the gravitational waves that we detect.
Second, gravitational waves can help us learn new things about the universe. You can think of it as a new era in astronomy, much like the one that the famous astronomer Galileo Galilei initiated 400 years ago, when he made a telescope and looked at the sky with it. We can use gravitational waves to look at the universe in a completely different way than we could before—using a “gravitational telescope.” Studying gravitational waves can help us better understand how cataclysmic (very powerful) astronomical events occur, such as collisions between black holes and neutron stars. This information could provide insights about events that occurred in the early stages of the universe’s formation, and could also help us find answers to intriguing questions about our own planet, such as how heavy elements like gold and platinum arrived on Earth3.
However, we are not yet very sophisticated in working with gravitational waves, so we usually combine the information that we get from measuring gravity with data that we already have from telescopes. This is enabling us to build a picture of cosmic events that goes well beyond what we could understand without the use of gravitational waves. In the future, as we get better at detecting gravitational waves, we hope to see cosmic phenomena by using only gravitational waves. These are very exciting times in cosmology, as our ability to detect gravitational waves opens a new window on cosmological events, which will help us to understand our universe better.
Recommendations for Young Minds
One of the lessons I have learned in life is that it is important to pay attention to your dreams and to try to fulfill them. Your dreams of the future tell you something about what you want in life—whether it is to be a physicist or an artist, or just to do something that is enjoyable, like travel or pursue a hobby that you love. You do not have to succeed in everything your dreams call you to do, but your dreams are telling you something about which routes to follow.
Another huge lesson that I have learned is that everything I do in my life is driven by one word: curiosity. Young people are naturally very curious, and you should treasure your curiosity and not let anything dim it—not your teachers, not your parents, nor anyone else. So, my advice is to pursue your curiosity, have fun, follow your dreams, and ignore everything that might limit your enthusiasm.
For those of you interested in science, science can be a lot of fun. There is nothing better in life than doing something good, having fun, and making a living. So, for me, science is a really good occupation. But you must remember that failure is a part of science and accept that not everything you do will succeed…and that failure can be a good thing. When you are at the forefront of science, doing something that has never been done before, it is frustrating at times. Each day, you will be in a situation where you do not really know whether you will make progress or perhaps even a new discovery, or whether you will do something that does not work at all. For individuals like me, this unknown contributes to the fun of doing science!
Glossary
Gravity: ↑ A force that causes objects to move toward one another.
Gravitational Waves: ↑ Disturbances in space and time that result from the motion of massive objects and spread as waves, at the speed of light.
Supernova: ↑ When a massive star gets old, it runs out of fuel, cools down, and collapses inward. This produces an enormous amount of energy, triggering nuclear fusion that leads to a massive explosion.
Nuclear Fusion: ↑ A reaction in which the nuclei of atoms fuse to create heavier nuclei, which releases a great amount of energy to the surrounding environment. The warmth and light of the Sun result from nuclear fusion.
Black Holes: ↑ The most massive objects known in the universe, where gravity is so strong that nothing, including light, can escape.
Neutron Stars: ↑ The remains of supergiant stars that collapse when they run out of fuel. They are typically only 10 km across and are extremely dense.
Proton: ↑ A positively charged particle present in the nucleus of all atoms. Protons are less than a billionth of the width of a human hair.
Interferometry: ↑ A measurement technique that uses laser beams to detect very small phenomena, such as gravitational waves in our case.
Conflict of Interest
The author declares that the research was conducted in the absence of any commercial or financial relationships that could be construed as a potential conflict of interest.
Acknowledgments
I wish to thank Noa Segev for conducting the interview which served as the basis for this paper, and for co-authoring the paper.
Footnotes
1. ↑To learn more about runaway, see Thermal runaway.
2. ↑To learn more about interferometry and how it is used to detect gravitational waves, see: Interferometer facts for kids or Gravitational Waves Explained Using Stick Figures. For more in-depth exploration of gravitational waves, see this introductory book. For a more advanced book on general relativity and gravitational waves, see this book or this one.
3. ↑We know that heavier elements can be made from lighter elements by nuclear fusion in stars. But, as we studied the lifecycle of stars, we saw that the heaviest element created that way is iron (atomic number 26). After the stars burn and use up all their iron, they collapse and do not continue producing heavier elements. Therefore, there should be another mechanism to create the heavier elements. Currently, the most widespread hypothesis is that heavier elements are created in the collisions of neutron stars, which are detectable using gravitational waves (for more information, see this MIT News article). We hope that, in the next years, enough data will be collected using the LIGO and Virgo detectors to validate this hypothesis with higher certainty.
References
[1] ↑ Newton, I. 1687. “Principia,” in The Principia: The Authoritative Translation and Guide, eds I. B. Cohen, and A. Whiteman (University of California Press). doi: 10.1525/9780520964815
[2] ↑ Einstein, A. 1915. Erklarung der Perihelionbewegung der merkur aus der allgemeinen relativitatstheorie. Sitzungsber. Preuss. Akad. Wiss. 47:831–9.
[3] ↑ Einstein, A., and Rosen, N. 1937. On gravitational waves. J. Frank. Inst. 223:43–54. doi: 10.1016/S0016-0032(37)90583-0
[4] ↑ Barish, B. C., and Weiss, R. 1999. LIGO and the detection of gravitational waves. Phys. Today 52:44–50. doi: 10.1063/1.882861
[5] ↑ Abbott, B. P., Abbott, R., Abbott, T. D., Abernathy, M. R., Acernese, F., Ackley, K., et al. 2016. Observation of gravitational waves from a binary black hole merger. Phys. Rev. Lett. 116:061102. doi: 10.1103/PhysRevLett.116.061102
[6] ↑ Abbott, B. P., Abbott, R., Abbott, T. D., Abernathy, M. R., Acernese, F., Ackley, K., et al. 2017. GW170817: Observation of gravitational waves from a binary neutron star inspiral. Phys. Rev. Lett. 119:161101. doi: 10.1103/PhysRevLett.119.161101
[7] ↑ Abbott, R., Abbott, T. D., Abraham, S., Acernese, F., Ackley, K., Adams, A., et al. 2021. Observation of gravitational waves from two neutron star–black hole coalescences. Astrophys. J. Lett. 915:L5. doi: 10.3847/2041-8213/ac082e