Abstract
One of the most interesting, exhilarating, and captivating questions that we can ask ourselves is: does life exist in other places in the universe? This question has sparked the imagination of many generations of science fiction authors, scientists, and intrigued citizens. In this article, I will tell you about the discovery of the first planet orbiting a sun-like star outside of our solar system (exoplanet), for which I was awarded a Nobel Prize in Physics in 2019. I will also tell you about the progress that was made since my discovery, and the current challenges we are facing when dealing with the question of discovering life elsewhere in the universe. How close are we to answering this long-standing question? Let us find out.
Professor Mayor won, jointly with Prof. Didier Queloz, the Nobel Prize in Physics in 2019 for the discovery of an exoplanet orbiting a sun-like star, and for his contributions to our understanding of the evolution of the universe and Earth’s place in the cosmos.
Can you imagine the possibility of other life forms living somewhere out there in the universe? At first, this thought may seem a little farfetched or hard to fathom. But, as an astrophysicist, I can tell you that it is actually quite likely. Why? Because there are so many planets in the universe—an unimaginable number—and some could be good candidates to support the formation of life. Before we dive into the possibility of other life in the universe, we will first take a look at how we discover planets outside our solar system.
How to Discover Distant Planets
When we search for habitable planets that could host life as we know it, we look for planets that are similar to Earth. One of the necessary conditions is that these planets should orbit around a star, which radiates heat and light. The star would then provide suitable temperature and energy-production conditions necessary for the development of life, as the Earth receives from the Sun. But the presence of a bright star (like the Sun) near a dim planet (like the Earth) does not allow scientists to detect the planet directly because the light reflected from the planet is outshone by the light from the bright star. For example, the Sun is about a billion times brighter than the light reflected from any of the planets orbiting it. Therefore, we need to develop indirect methods to detect the presence of a planet. One of these methods involves detecting the changes that this planet causes on the velocity of the nearby star. To understand this method, we must become familiar with two concepts—spectral lines and the Doppler effect.
Spectral Lines
As you might know, each atom has energy levels that correspond to the movement of electrons around its nucleus. When light passes through an atom, some of the light’s wavelengths, corresponding to the energy levels of the atom, get absorbed by the atom. This means that, if we can detect the emitted light after it interacts with an atom, we get a specific “fingerprint” of that atom from the specific wavelengths we observe. The detected spectrum of light, which was previously continuous, is now composed of lines of reduced (dark) or intensified (bright) light at specific wavelengths. These lines are called spectral lines1.
Spectral Lines from Distant Stars
Each star has a specific combination of atoms in its surrounding atmosphere. So, when we detect the star’s light after it passes through the star’s atmosphere, we get its unique fingerprint of spectral lines, resulting from all the different atoms in the star’s atmosphere. We can use small shifts in these spectral lines to infer the presence of a planet orbiting around this star. These small shifts are due to a phenomenon called the Doppler effect.
The Doppler Effect
Have you ever noticed that, when an ambulance with its siren on moves toward you, the siren’s pitch changes—getting much higher, and more shrill, as it approaches and then dropping in pitch and becoming lower as it passes you? Actually, the sound coming out of the siren does not change. What does change is that, when the ambulance approaches you, each sound wave takes less time to reach you than the previous wave, which causes an increase in the frequency of the waves. This makes the siren sounds faster when it approaches you and slower when it moves away from you2 (Figure 1). This shift in the observed frequency is called the Doppler effect.
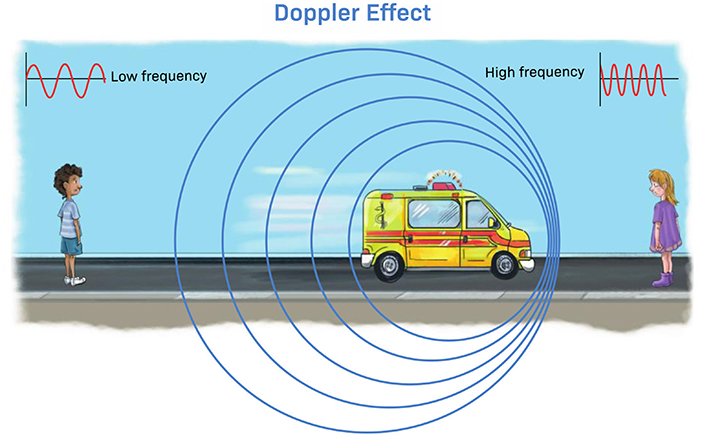
- Figure 1 - The Doppler effect.
- When an ambulance with a siren moves toward you (person on the right), the siren’s sound reaches you more quickly (with a higher frequency) than when it moves away from you (person on the left, low frequency). This effect is due to the change in the frequency from the point of view of the observer. In reality, the frequency of the siren does not change.
The same is true for any type of wave, including light. So, when a shining object like a star moves toward us, its spectral line image will shift to shorter wavelengths and higher frequency (called blue shift), and when it moves away from us, the spectrum will shift toward longer wavelengths and lower frequency (called red shift). Now, when a planet orbits around a star, it influences the movement of the star due to the planet’s gravity—the star moves along an elliptical path caused by the orbit of the planet, so at some points in time the star will move toward Earth and at other times it will move away from Earth. This change in the velocity of the star relative to Earth will cause a change in the star’s spectral lines3. Overall, this means that we can indirectly infer the presence of an orbiting planet around the star by measuring the Doppler shift in the spectral lines of the star (Figure 2).
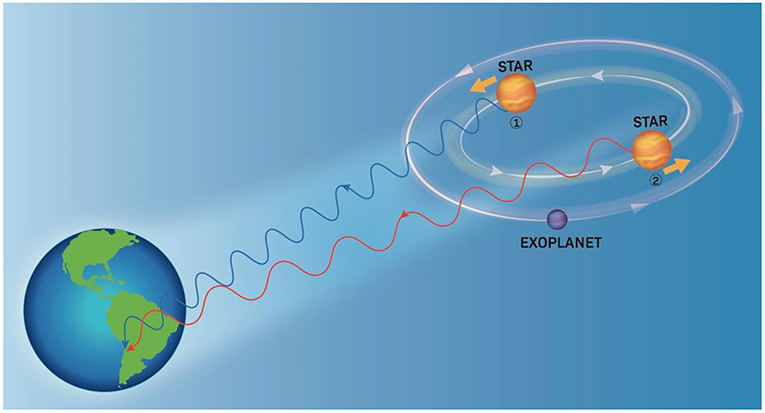
- Figure 2 - Detecting an exoplanet using the Doppler effect.
- An unseen exoplanet orbiting around a distant star causes the star to move along an elliptical path. The star will sometimes be moving toward Earth (1) and sometimes away from Earth (2). Due to the Doppler effect, we see shifts in the frequency of the spectral lines emitted by the star, which will be higher (blue) when the star is moving toward the Earth and lower (red) when moving away from it. This shift can be used to infer the presence of the exoplanet (Figure revised from ESO).
The Cross-Correlation Technique
There is a big challenge in terms of using the Doppler effect to detect the presence of an unseen planet. The shifts in the star’s velocity caused due to the exoplanet are in the range of only a few meters per second, or even less. In terms of Doppler shifts of a star’s spectral lines, this small shift in the star’s velocity means shifts of <1 billionth (1/1,000,000,000) of its emitted wavelength [1]. This is such a small fraction that it is impossible to precisely measure when using changes in only a single spectral line due to the Doppler effect.
So, what did we do to increase the precision of this measurement? We used another clever trick, called the cross-correlation technique, which was optimized in the 1980s and 1990s and played a big part in allowing us to detect planets outside our solar system.
The key idea here is that, instead of measuring the shift in only one spectral line emitted from a star of interest, we measured the collective shift due to the Doppler effect on all the spectral lines emitted by the star. We did this by using a device called a CORAVEL spectrometer (Figure 3A) [1, 2]. The CORAVEL spectrometer contains a plate with a set of holes (Figure 3B), which are located exactly at the positions where we expect to have dark spectral lines in the light coming from a particular star. All the transmitted light emitted through these holes is sent to a single detector. When the dark spectral lines of the star are exactly in front of the holes in the plate, we detect a minimum of the transmitted light (Figure 3C, left). However, if we have a Doppler shift due to the exoplanet influencing the movement of the star, then the position of many thousands of spectral lines will shift simultaneously relative to the position of the holes on the plate, and the amount of light transmitted through the holes will increase (Figure 3C, right). After this Doppler shift, we need to move the plate so that the holes are again lined up with the black spectral lines, so that we again obtain minimum light in our detector.
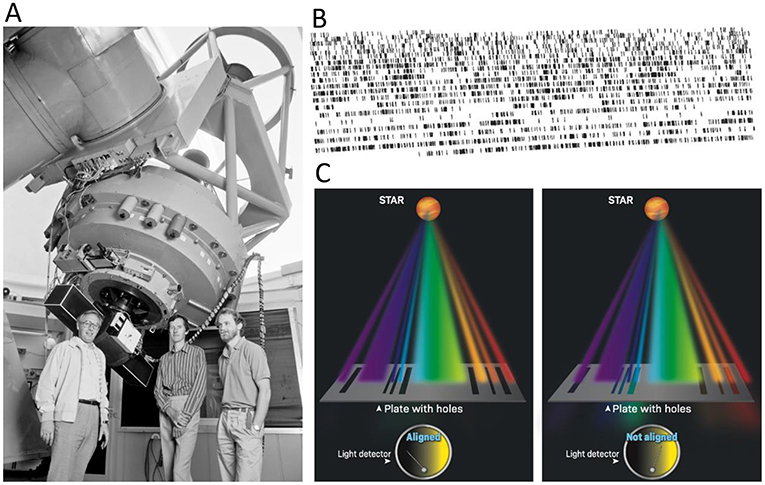
- Figure 3 - Cross-correlation measurement with the CORAVEL spectrometer.
- (A) Staff members standing in front of the CORAVEL spectrometer, located in the La Silla Observatory in Chile. (B) The original CORAVEL plate with its holes (black stripes) that we used to detect the Doppler shifts of many (dark) spectral lines arriving from 51 Pegasi, using the cross-correlation method. (C) The light coming from a star is concentrated by the CORAVEL telescope and projected onto a plate with holes. When the black lines are aligned with the holes in the plate, then the minimum amount of light reaches the light detector (left, ”Aligned“). When the black lines are shifted due to the Doppler effect, as the result of the presence of a planet orbiting around this star, they are no longer aligned with the holes and a larger amount of light passes the plate and arrives at the detector (right, ”Not aligned"). This shift in the location of the spectral lines, enables us to infer the presence of a planet orbiting around the star (Image credits: (A) ESO and (B) reference [1]).
Therefore, when we measure the star’s absorption spectral lines at two positions on its trajectory, and move the plate so that the minimum light is detected each time, we know how much the plate was moved between the first minimum (first position of the star) and the second minimum (second position of the star). This shift in the plate’s position between two minima is the direct result of the Doppler shift of the spectral lines of the star, due to the presence of the exoplanet. By calculating the Doppler shift in the star’s spectral lines, in combination with other measurement, we can learn about the characteristics of the detected exoplanet.
The cross-correlation technique enabled us to concentrate the Doppler information from all the individual spectral lines into one single quantity. We call this quantity the Doppler velocity, as it tells us the change in the velocity of the star due to the presence of the nearby orbiting planet. Using Doppler velocity, combined with some other measurements, we can infer not only the presence of the planet, but also learn about its mass, size, and the time it takes for the planet to complete one orbit around the star. This method enabled us to detect 51 Pegasi b—the first exoplanet that my colleague Didier Queloz and I discovered in 1995 [3]. With recent spectrographs, the stellar spectra is obtained in a somewhat different way. Instead of scanning the spectrum on a physical plate, the spectrum is recorded on special sensors called CCD detectors (like we have in digital cameras). Then, it is analysed by a computer, based on the same cross-correlation principle that we have seen above.
51 Pegasi b: The Discovery of the First Measured Exoplanet Orbiting Around a Sun-Like Star
51 Pegasi b (Figure 4A) is a planet located about 50 light-years (about 4.7 hundred-thousand billion kilometers!) away from Earth, in the Pegasus constellation in the Milky Way4. Its temperature is hot, about 1,000 degrees Celsius. It orbits around a Sun-like star, called 51 Pegasi, and completes its orbit about every 4.2 days. 51 Pegasi b is composed mostly of gases, and is classified as a gas giant, like Jupiter. Because it orbits so close to its star, it is sometimes referred to as a “hot Jupiter.” 51 Pegasi b is about 47% lighter in mass and 50% larger in size than Jupiter. The 51 Pegasi star is about 11% heavier and 23% larger than our Sun.
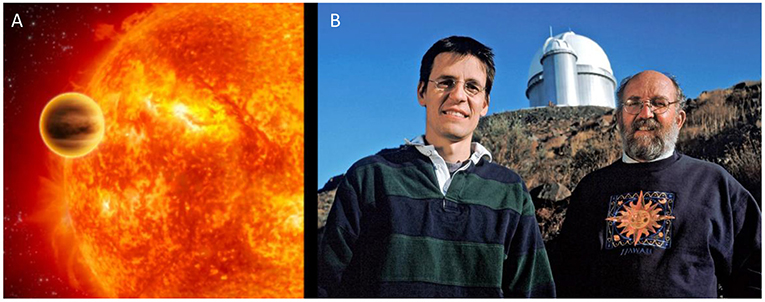
- Figure 4 - (A) An artistic representation of 51 Pegasi b exoplanet (small sphere) and the star it orbits, the 51 Pegasi star.
- Pegasi 51 b is a gaseous planet about 50 light years away from Earth. It is the first planet outside our solar system that was found orbiting around a Sun-like star. (B) My colleague, Didier Queloz (left) and me, standing in front of the 3.6-m HARPS telescope at La Silla observatory in Chile. Since 2003, the HARPS spectrograph, which implements the cross-correlation technique that we developed, is used to search for exoplanets [Image credits: NASA/JPL-Caltech (A) and L. Weinstein/Ciel et Espace Photos (B)].
As mentioned above, 51 Pegasi b was the first exoplanet discovered orbiting around a star. While this star and exoplanet are themselves fascinating to study, their discovery also provided a breakthrough in the field of planet detection, in two significant ways. First, the discovery of 51 Pegasi b proved that planets orbiting stars exist in other places in the universe besides our solar system—something that was not definite before—and that these planets can be detected using the cross-correlation technique. Second, it proved a hypothesis called planetary migration, which is the idea that, over time, planets can migrate, or move, closer to the stars they orbit5. Giant planets that are very close to the stars they orbit are very attractive for astrophysicists because such planets can be discovered in a shorter time period using the cross-correlation technique. Prior to the discovery of 51 Pegasi b, scientists believed that the orbit period of a giant star could not be shorter than 10 years, which meant that it would take 10 years to detect one planet using the Doppler effect! But our discovery showed that the orbit period could be as short as a few days—a thousand times shorter than was expected! This means some exoplanets could be detected in just a few days.
Both of these breakthroughs contributed significantly to the detection of additional star-orbiting exoplanets. Today, more than 5,000 such planets have been discovered! This is an important step toward finding possible life in the universe.
Life In the Universe
Our current definition of life as we know it includes three main characteristics: a living system should be able to protect itself from the environment, interact with the environment, and pass its information to the next generation. This passing of information is performed using long chains of atoms and molecules (called the genetic material, or the DNA), which are very fragile. DNA molecules require specific temperatures and the presence of water. This means that, if there is an exoplanet with life on it, it must fulfill these requirements6. Now, how likely is it to find such a planet? Well, since there are so very many planets in the universe, we are absolutely sure that many of them have the possibility for life to develop. But, as scientists, we are not satisfied with simply saying “yes, it is likely”—we want to prove it directly.
It might seem that the simplest way to discover life on other planets would be to send spacecrafts to them, to look around and take pictures. But this is impossible with our current technology and current understanding of physics, because it would take far too long for a spacecraft to get to these very far planets and it would require an unreasonable amount of energy7. This means that we need to use remote detection methods, which are indirect measurements and observations that would imply the probable existence of life on a certain planet. For example, we could analyze the chemical components in the atmospheres of exoplanets using spectral lines. Since we are very familiar with the spectral lines of the chemical components in Earth’s atmosphere, such as oxygen (ozone), nitrogen, methane, and carbon dioxide, we can try to find similar patterns in the atmospheres of other planets8. This and other directions of research, though promising, are very complicated and require further development before they become useful. So, the big questions of whether and how can we detect life on exoplanets remain a marvelous challenge for the next generation of young scientists—like you!
Recommendations for Young Minds
To be a scientist, I believe that you need to have a lot of curiosity. Science is not a ”normal“ job, it is not only done to earn money. But if you are curious about any topic in science, I believe you will be happy as a scientist—it is that simple. I have never regretted choosing to be a scientist. For me, one of the pleasures of being a scientist is that you have the privilege of working with people from all over the world. It is nice to feel that you have friends in many places around the globe.
I also believe it is very important for scientists to be able to work well in teams. I have been leading several research groups for many years, and I noticed that even if only one person does not mix well with the team, the whole team can be negatively affected. As part of a team, you should be comfortable with your colleagues and love to go to work with them. So, make sure to match up with the right people, and enjoy your everyday interactions.
Additional Materials
- Michel Mayor, Nobel Prize in Physics 2019: Official interview (Nobel Prize).
- The 2019 Nobel Prize in Physics—The discovery of the first exoplanet (Nobel Prize).
Glossary
Habitable Planet: ↑ A planet that has the conditions necessary to support life.
Spectral Line: ↑ A line of light at a specific wavelength, which is either absorbed by or emitted from atoms.
Doppler Effect: ↑ A physical effect in which the measured wavelength (frequency) of a wave changes as its source moves toward or away from the observer.
Cross-Correlation Technique: ↑ A method that uses the Doppler effect on spectral lines from the light of a distant star to detect planets outside our solar system.
Spectrometer: ↑ A device used to detect and analyze the spectrum of light, in our case light from stars and planets.
Doppler Velocity: ↑ The change in the velocity of a star resulting from the presence of a close orbiting planet.
Exoplanet: ↑ A planet that is located outside our solar system.
Conflict of Interest
The author declares that the research was conducted in the absence of any commercial or financial relationships that could be construed as a potential conflict of interest.
Acknowledgments
I wish to thank Noa Segev for conducting the interview which served as the basis for this paper, and for co-authoring the paper. Thank to Sharon Amlani for providing Figures 1, 2, 3C.
Footnotes
1. ↑To learn more about spectral lines, see here.
2. ↑See demonstration here and explanation in Figure 1.
3. ↑See a video demonstration here.
5. ↑For more information about planetary migration, see here.
6. ↑If a planet does satisfy these requirements, it does not necessarily mean that there is life on it, only the potential for life. We could also dream about completely different life forms that could develop under conditions different from those we know, but we start with the simplest options based on current science.
7. ↑This also means that, even if we find a habitable planet outside our solar system, it will not be suitable for human immigration with our current understanding of physics. Therefore, we should make great efforts to protect Earth so that it stays habitable for humans for many generations to come.
8. ↑For more information about this method, you can read this paper [4].
References
[1] ↑ Mayor, M. 2020. Nobel lecture: plurality of worlds in the cosmos: a dream of antiquity, a modern reality of astrophysics. Rev. Mod. Phys. 92:030502. Available online at: https://journals.aps.org/rmp/abstract/10.1103/RevModPhys.92.030502
[2] ↑ Baranne, A., Mayor, M., and Poncet J. L. 1979. Coravel-a new tool for radial velocity measurement. Vist. Astron. 23:279–316. doi: 10.1016/0083-6656(79)90016-3
[3] ↑ Mayor, M., and Queloz, D. 1995. A Jupiter-mass companion to a solar-type star. Nature 378:355–9.
[4] ↑ Schwieterman, E. W., Kiang, N. Y., Parenteau, M. N., Harman, C. E., DasSarma, S., Fisher, T. M., et al. 2018. Exoplanet biosignatures: a review of remotely detectable signs of life. Astrobiology. 18:663–708. doi: 10.1089/ast.2017.1729