Abstract
Since the beginning of my career, I have been fascinated by the phenomenon of gravitational waves—ripples in space and time that spread at the speed of light. At first, my interest was simply in understanding the concepts, but once I realized there was a possibility to actually detect gravitational waves, I was hooked. I decided to join the efforts to design and build gravitational wave detectors, and I worked on that challenge for a few decades until the first successful detection in 2015. In this article, I will tell you about how a gravitational wave detection instrument called LIGO came about, how my students and I helped improve it, and what the future holds for LIGO and for other gravitational wave detectors that could revolutionize our understanding of the universe.
As background materials for this article, it is recommended to view a previously published article on gravitational waves by the Nobel Laureate Prof. Barry Barish.
Professor Kip S. Thorne won the Nobel Prize in Physics in 2017, jointly with Profs. Rainer Weiss and Barry Barish, for decisive contributions to the LIGO detector and the observation of gravitational waves.
How LIGO Came About
Gravitational waves are disturbances in the fabric of space and time (Figure 1). When an extreme astronomical event occurs in the universe, such as a collision between two black holes, it creates “ripples” in space itself, which spread throughout the universe at the speed of light. I have been intrigued by gravitational waves since I was a young researcher. Already in the mid-1960s, I was working on the theory of gravitational waves and the sources of those waves. Initially, my goal was to understand how gravitational waves are emitted and how does this emission process affect the source that emits them. But then, in 1969, a colleague of mine named Joseph Weber announced that he might have detected gravitational waves [1]. Although after a few years it became clear that Weber was not actually seeing gravitational waves, I got hooked by the questions of whether there was a way to detect these waves that had a good probability of success, and what we could learn from the waves if we did succeed.
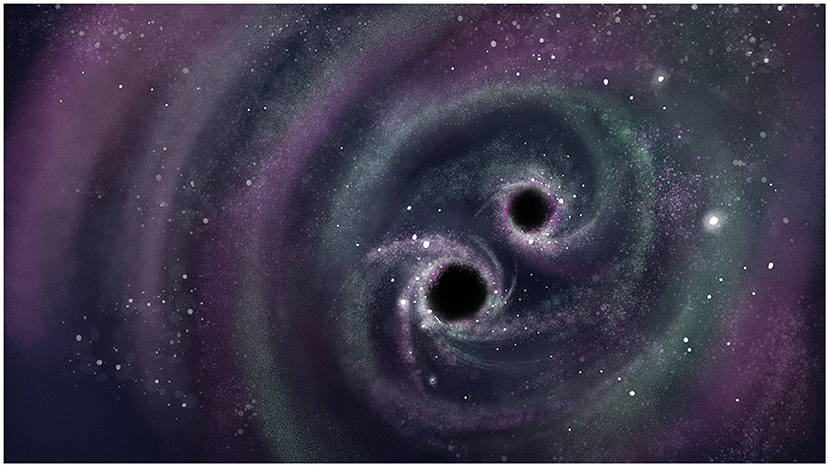
- Figure 1 - Gravitational waves.
- An artistic representation of gravitational waves spreading as “ripples” in space around two massive, moving objects (e.g., black holes).
What excited me most was the opportunity to create a new scientific field—gravitational wave astronomy—that could become a powerful tool for exploring the universe in the coming decades and centuries. I realized that gravitational wave astronomy would give us a new “window” through which we could observe the universe, and it could potentially revolutionize our understanding of how the universe works. The ability to detect gravitational waves would allow us to study a huge range of phenomena that we could not previously study at all, or could not study adequately [2], including the properties of black holes, supernovae, and the origins of the universe.
In 1972, another colleague of mine named Rainer Weiss proposed a new method for detecting gravitational waves, based on laser measurements [3]. I was very skeptical at first, but after 3 years of discussion and study I became convinced that it could succeed; and so I decided that, as a theorist, I would devote much of the rest of my career to helping Weiss and his experimental physicist colleagues achieve success. Using our knowledge of the expected properties of gravitational waves, we estimated that we could close the remaining technological and scientific gaps and build a successful detection technology within roughly 20 years. It ultimately took us about 40 years to build the Laser Interferometer Gravitational-Wave Observatory (LIGO) and to detect the first-ever-measured gravitational waves in 2015 [4]—but it was worth the effort.
Contributing to LIGO
The LIGO detector contains a laser that emits a light beam toward an element called a beam splitter (Figure 2). The beam splitter splits the light into two perpendicular routes called the arms of the detector, which contain mirrors that reflect the light beam back and forth between them hundreds of times. The two arms’ beams leak out through their input mirrors then interfere in the beam splitter to produce an output light signal in a photon detector. LIGO works on the principle that, when a gravitational wave hits it, the wave squeezes one arm of the detector and lengthens its other arm, and vice versa, in an alternating fashion, causing the output light beam to rise and fall in intensity (to see a simulation of this process, watch this video). To measure the gravitational wave, we must be able to detect extremely tiny changes in the length of the detector’s arms. In fact, for the strongest gravitational waves that LIGO detects, the change we need to measure is 1021 times smaller than the length of the arm itself. In LIGO the arms are 4 km long, so we must be able to detect changes on the order of 4 × 10-18 meters—that is about 1,000 times smaller than the nucleus of an atom!
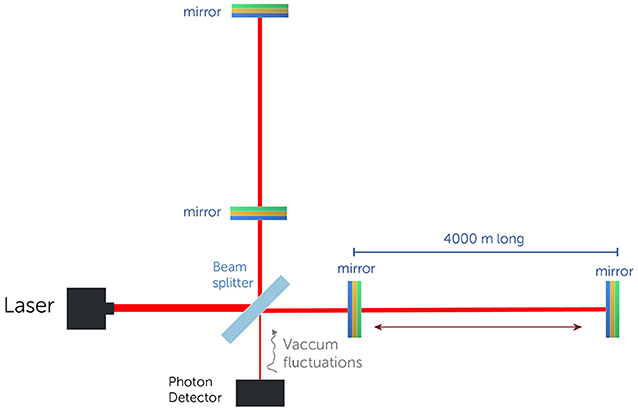
- Figure 2 - Vacuum fluctuations in the LIGO interferometer.
- When my students and I worked on LIGO, we identified sources of noise that affected the detector’s measurements. One of these sources, found by my student Carlton Caves, was fluctuations of the electromagnetic field that we call vacuum fluctuations. You can think of this as disturbances that enter the detector “backwards” and superpose on the laser light beams in the two arms of the detector, causing the beam intensity to fluctuate up in one arm and down in the other, and vice versa.
A big part of the work my students and I did for the LIGO detector was to predict and address current and future problems with detection sensitivity. We primarily wanted to understand noise (errors caused mainly by undesired movements of different elements in the detector) and find ways to reduce the noise as much as possible. One serious source of noise—a source of which LIGO’s scientists were oblivious until a student of mine named Yuri Levin pointed it out—is the coatings we used on our mirrors (the colorful rectangles in Figure 2). When light bounces off a regular mirror, some of it is reflected and some of it passes through the mirror. To maximize the amount of light that is reflected from LIGO’s mirrors—so that we will have the strongest possible signal arriving at our photon detector, the experimenters coat them with alternating thin layers of two very different dielectric materials; the thickness of each layer must be the wavelength of the laser’s light (can you figure out why?). To make LIGO’s measurements as accurate as possible, we wanted the light beam in each arm to bounce back and forth as long as a half period of the longest-period gravitational waves we seek, which means a few hundred bounces (trapping the light longer does not gain us anything; can you figure out why?). To achieve hundreds of bounces, we used more than a dozen coating layers.
My student Yuri Levin figured out that, at room temperature, vibrations of these coatings produce serious thermal noise—a big surprise to the experimenters. Although the amplitudes of the coating vibrations may seem extremely tiny—on the order of 10-15 meters—they are huge considering we wanted to measure changes of about 10-18 meters in the positions of the mirrors. Levin discovered the severity of coating thermal noise by first inventing a very clever new way to figure out how much thermal noise comes from various parts of the detector [5] (its mirror coatings, the wires by which the mirrors hang, the fused silica of the solid mirrors themselves, …). Levin’s work paved the way for other scientists to address other sources of thermal noise—some of which, like mirror-coating noise, LIGO scientists were previously completely unaware of.
Another of my theoretical physics students, Carlton Caves, revolutionized our understanding of quantum noise in LIGO’s detectors. Quantum noise comes from random fluctuations that are an omnipresent, irremovable, fundamental feature of our universe. In LIGO, before Caves, we knew two types of quantum noise: the first is random fluctuations in the arrival of the light beam’s photons at the photon detector that measures them; the second was random fluctuations in the photons’ bouncing off the mirrors and thereby making the mirrors’ positions fluctuate randomly [6]. The intriguing part was that each of these two noises had to arise from differences between the ways photons behaved in LIGO’s two arms (otherwise, the detector would not have spotted the noise as it would cancel out). We could not understand what causes these differences in photon behavior, until Caves figured it out [7]. Caves realized that the source of the two types of noise was a phenomenon called vacuum fluctuations (Figure 2), which are inherent fluctuations of the electromagnetic field that remain when everything else has been removed, i.e., that remain “in vacuum”. It turned out that the noise-producing vacuum fluctuations entered our system “backwards”, from the photon detector into the arms of LIGO. They superimposed on the laser light in the two arms oppositely: when making the total light intensity fluctuate up in one arm, they make that in the other fluctuate down. This was the cause of LIGO’s weird quantum noise. To reduce this quantum noise, Caves conceived a sophisticated method, called squeezing the vacuum [7], which has become a foundation for a whole new technology called quantum precision measurement, that today is playing a major role in LIGO [8].
Future Plans for Gravitational Wave Detectors
In our work on LIGO, we had a constant stream of challenges to address. Much of our learning happened as we went along, while we kept trying to improve our detectors. Our initial LIGO detectors reached the apex of their performance in 2010—sufficient to see neutron stars spiraling together out to about 50 million light years from Earth—but we saw no sign of any gravitational waves. In 2008, we started working on the next generation of LIGO detectors, called Advanced LIGO. As one major improvement, my LIGO colleagues changed the way the mirrors were hanging, to reduce the influence of Earths’ vibrations on the detectors, while also reducing the thermal noise of the wires themselves (Figure 3A). They also incorporated better mirror coatings that produce less thermal noise and have better reflectivity. These and many other improvements reduced the noise sufficiently, by September 2015, for the advanced detectors to see 5 time farther than the initial detector had (and so observe a volume of universe 53 = 125 times larger than in 2010). This was sufficient to bring our wonderful first discovery of gravitational waves. Further improvements, including Caves’ squeezing-based quantum precision measurement technology, have brought LIGO from seeing about one black hole collision every 6 weeks in 2015 to about one every 3 days in 2023. And I estimate several each day by the late 2020s. This will be roughly a 100-fold improvement over 2015!
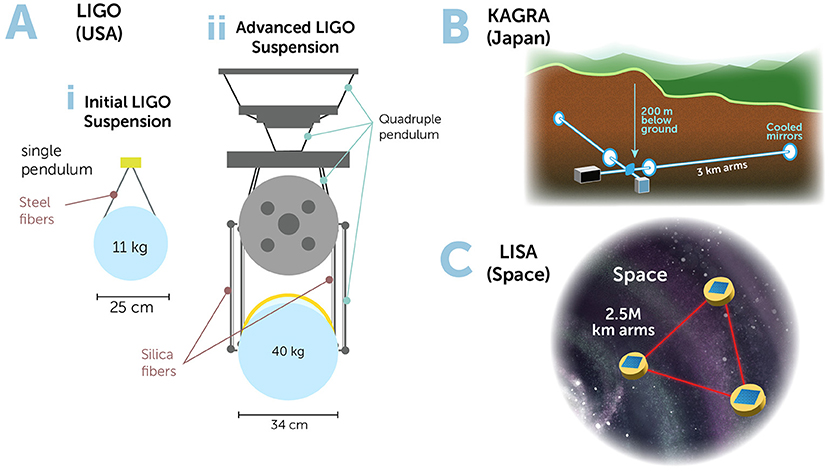
- Figure 3 - Advanced LIGO and other detectors.
- (A) (i) In our initial LIGO system, mirrors were hanging from steel fibers as a single pendulum. (ii) In advanced LIGO, mirrors were hanging from silica fibers connected to four different pendulums. This significantly decreased the noise coming from Earth’s vibrations. (B) A similar type of detector called KAGRA started operating in Japan in May 2023. KAGRA is located 200 meters underground and its mirrors are cooled to -250°C to reduce thermal noise. (C) LISA is a future space-based detector planned by the European Space Agency for operation in the late-2030s.
Another LIGO project called LIGO India was approved in 2016 and is expected to be fully operational by 2030. Having this third LIGO site in India should improve our ability to figure out where gravitational waves come from. By analyzing the differences of wave-arrival times at the various detectors (LIGO’s three in the United States and India, a fourth called Virgo in Italy and a fifth in Japan called KAGRA), we can deduce the location on the sky from which the waves came.
The VIRGO detector (originally an Italian-French collaboration, with Netherlands, Poland, Hungary and Spain now collaborating) was completed in 2003. It started making observations in 2017, and together with LIGO in August 2017 discovered the first collision of two neutron stars. KAGRA in Japan (Figure 3B), whose construction started in 2010, is located underground, and its mirrors are cooled to -250°C to reduce thermal noise. Its first successful observations started May 25, 2023. LIGO, VIRGO, and KAGRA all have arms that are 3 or 4 kilometers in length, and they can measure gravitational waves with similar frequencies, in the range of about 10–1,000 Hertz (Hz). There is a plan for building two much larger ground-based gravitational wave detectors that will be able to detect far weaker waves than LIGO, Virgo, and KAGRA. These projects are called the Einstein Telescope (to be built in Europe with 10 km arms) and the Cosmic Explorer (to be build in North America with 40 km arms). They are expected to be operational in the late 2030s.
A different kind of gravitational wave detector is planned to operate in space starting in the late 2030s. This project is called LISA and it will be constructed and operated by the European Space Agency (Figure 3C). LISA is planned to have extremely long arms: 2.5 million km! This and its great distance from our noisy earth will allow it to measure gravitational waves with much lower frequencies, from about 0.1 mHz-1 Hz (1 mHz is Hz). Similar space-based projects, called TianQin and Taiji, are planned by China and like LISA are planned for operation in the 2030s.
Studying the Universe With Gravitational Waves
The most exciting thing about gravitational waves is that they can teach us about the nature of space and time, about the properties and behavior of black holes and other phenomena made wholly or partially from warped spacetime (“the warped side of our universe”), and even about the origins of our universe. One of the most intriguing questions for me personally is: What are the details of our universe’s big-bang birth and of the ill-understood laws of quantum gravity that governed the big bang? Quantum physics guarantees that some gravitational waves—at least gravitational vacuum fluctuations—emerged from the big bang and carry information about its details. We physicists are fairly sure that those primordial waves (or fluctuations) were greatly amplified by an early, very rapid “inflationary” expansion of our universe, to produce strong enough gravitational waves for detection in the next several decades by two different kinds of detectors: a follow-on successor to LISA, and polarization of cosmic microwaves. I am optimistic that these will both succeed in detecting primordial gravitational waves, and that those observations will play a major role in pinning down details of the Big Bang and of the laws of quantum gravity—though perhaps not until the middle of this 21st century. This may usher in a marvelous new revolution in our understanding of our universe.
I want to end by passing on a piece of advice that I got as a kid. When I was 4 years old, my grandfather told me that, if I grow up to have a job that feels like play, then I may experience great success in life. If it feels like play, I will work very hard at it, and that pleasureful work will really pay off. I listened to him and chose physics as my profession. For me, physics is like play, I have enjoyed it enormously, and have found some success. So, my advice to you is: try to find a career that is both meaningful to you, and that you also really love. That love will then give you the strength needed to work hard enough to become truly successful.
Glossary
Noise: ↑ Errors in measurement that are caused by fluctuations of various elements in the detector such as mirror coatings, wires by which mirrors hang, and photons in the detector’s light beams.
Thermal Noise: ↑ Fluctuations (tiny movements) of matter that result from heat.
Quantum Noise: ↑ Noise arising from the random, un-removable fluctuations that quantum theory imposes on everything.
Black Hole: ↑ An object, made from warped spacetime, that has such a strong force of gravity that nothing which falls through its surface (its horizon) can ever get back out.
Conflict of Interest
The author declares that the research was conducted in the absence of any commercial or financial relationships that could be construed as a potential conflict of interest.
Acknowledgments
I wish to thank Or Raphael for conducting the interview which served as the basis for this paper and for co-authoring the paper, and Alex Bernstein for providing the figures.
References
[1] ↑ Weber, J. 1969. Evidence for discovery of gravitational radiation. Phys. Rev. Lett. 22:1320. doi: 10.1103/PhysRevLett.22.1320
[2] ↑ Press, W. H., and Thorne, K. S. 1972. Gravitational-wave astronomy. Ann. Rev. Astron. Astrophys. 10:335–74. doi: 10.1146/annurev.aa.10.090172.002003
[3] ↑ Weiss, R. 1972. Electronically Coupled Broadband Gravitational Antenna. Quarterly Progress Report of the Research Laboratory of Electronics, Massachusetts Institute of Technology, No. 105, 54.
[4] ↑ Abbott, B. P., Abbott, R., Abbott, T. D., Abernathy, M. R., Acernese, F., Ackley, K., et al. 2016. GW150914: the advanced LIGO detectors in the era of first discoveries. Phys. Rev. Lett. 116:131103. doi: 10.1103/PhysRevLett.116.131103
[5] ↑ Levin, Y. 1998. Internal thermal noise in the LIGO test masses: a direct approach. Phys. Rev. D 57, 659. doi: 10.1103/PhysRevD.57.659
[6] ↑ Thorne, K. S. 2018. Nobel lecture: LIGO and gravitational waves III. Rev. Mod. Phys. 90:040503. doi: 10.1103/RevModPhys.90.040503
[7] ↑ Caves, C. M. 1981. Quantum-mechanical noise in an interferometer. Phys. Rev. D 23:1693. doi: 10.1103/PhysRevD.23.1693
[8] ↑ Ganapathy, D., Jia, W., Nakano, M., Xu, V., Aritomi, N., Cullen, T., et al. (LIGO O4 Detector Collaboration) 2023. Broadband quantum enhancement of the LIGO detectors with frequency-dependent squeezing. Phys. Rev. X 13:041021. doi: 10.1103/PhysRevX.13.041021