Abstract
The retina is the thin layer in the back of the eyeball where vision begins, and it is actually considered part of the brain. Despite its small size, nerve cells in the retina carry complex information about the colors, edges, and motion that we see. The retina is easier to study than other brain areas, so not only can retinal research help us understand vision, it can even teach us how other brain areas work. The brain is adaptable—it can change its activity as changes happen in the environment, but the activity of retinal cells was thought to be fixed and stable even in the presence of changes in the visual environment of the animal. In our research, we discovered that the retina, too, can change its activity. Therefore, retinal research can teach us about other brain areas, including how they adapt to changes in the environment.
The Brain—A Complex Organ
The brain is where information about everything we feel, think, and do is processed. For thousands of years, scientists and philosophers have been trying to understand how the brain works and how our senses allow us to understand the world around us. Today, we know that the brain works by means of nerve cells, called neurons, that send messages in the form of electrical signals. The place where two neurons connect to each other to send and receive messages is called a synapse.
In the laboratory, we can see various areas of the brain and even measure their electrical activity, but we still do not fully understand how the activity of neurons represents the world around us. For example, how can the brain tell the direction and speed of a moving car? How does it distinguish between the letters A and B?
One reason that it is difficult for researchers to understand brain activity is that the brain has an enormous number of neurons. Each neuron can send and receive electrical signals to and from thousands of other neurons, which makes understanding neuron activity extremely complex. For this reason, scientists sometimes focus on small groups of neurons, called neural networks. By learning how neural networks work, researchers can understand more about the whole brain.
Cells in the Retina Have Specific Roles
In our laboratory we study vision—we ask how the brain responds to the things we see around us. We research the retina, which is the “first stop” in vision. The retina, a thin layer of neurons located at the back of the eyeball, is actually a part of the brain (Figure 1A).
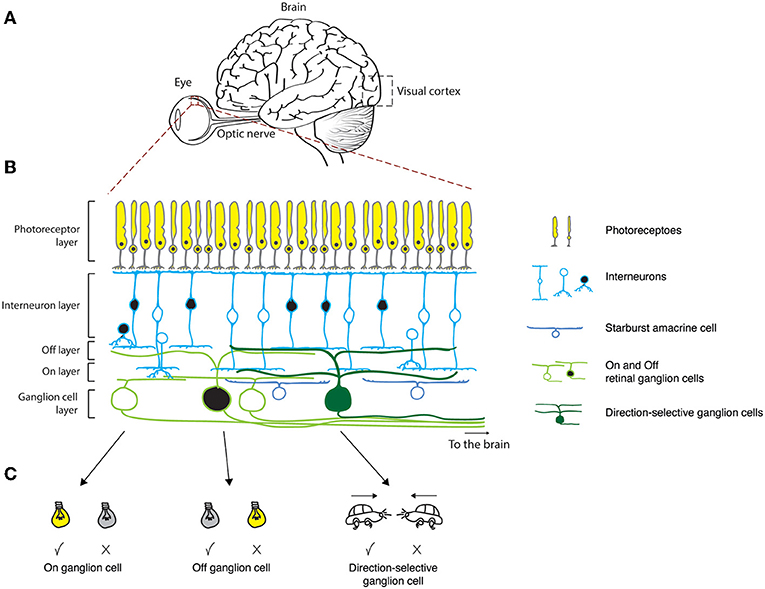
- Figure 1 - (A) Side view of the eye and the brain.
- (B) Layers of cells in the retina. The first layer contains the photoreceptors, the next layer contains the interneurons, and the final layer contains the ganglion cells. (C) Each ganglion cell type responds differently to visual stimuli (The check mark indicates that the cell responds, and the x indicates no response.). On ganglion cells respond to light onset; Off ganglion cells respond to light offset; direction-selective ganglion cells respond to movement in a certain, preferred, direction.
What kinds of cells make up the retina, and how do they work together to enable vision? The first retina layer contains light-sensitive cells called photoreceptors (Figure 1B). When a beam of light hits the photoreceptors, a sequence of biochemical reactions causes an electrical reaction. Thus, photoreceptors turn light into electrical signals. The electrical signals are then passed on to the second retina layer, made of interneurons, and from there to the last layer, where the ganglion cells are located. Ganglion cells send long projections called axons deep into the brain. These axons carry electrical messages to the parts of the brain that process visual signals—telling them about what the eye is seeing.
There are several types of ganglion cells, each with their own function [1]. For example, there are ganglion cells that respond to light onset (called On ganglion cells), some that respond to light offset (called Off ganglion cells), and even some that respond to movement in a certain direction (called the preferred direction, Figure 1C). Cells of this last type are called direction-selective ganglion cells. For example, there are direction-selective ganglion cells that respond only when we see something moving to the right. These cells will send electrical signals to the brain in response to a car passing in front of us from left to right (Figure 1C), or if we are watching a tennis match and the ball comes from our left to our right. But if the car turns around and heads to the left, or if the tennis player hits the ball back to the player on our left, these same cells will not respond. Instead, there are other ganglion cells that respond to movement toward the left, and still others that respond to up and down movements.
Connections Between Retinal Cells Determine Their Responses
How can ganglion cells act in such different and specific ways? The answer lies in the interneurons that pass on electrical signals from the photoreceptors to the ganglion cells. There are more than 60 different types of interneurons, and a ganglion cell’s response depends on which type of interneuron connects to it. The connectivity between the interneuron and the ganglion cell—such as its location, strength, and so on—determines the response of the ganglion cell.
For example, how do direction-selective ganglion cells know what direction to respond to? Studies have found that a type of interneuron called a starburst amacrine cell helps the ganglion cell to do this. Starburst amacrine cells are connected to direction-selective ganglion cells on one side only. For example, a ganglion cell that receives connections from a starburst amacrine cell on its right will respond to motion to the right (Figure 2).
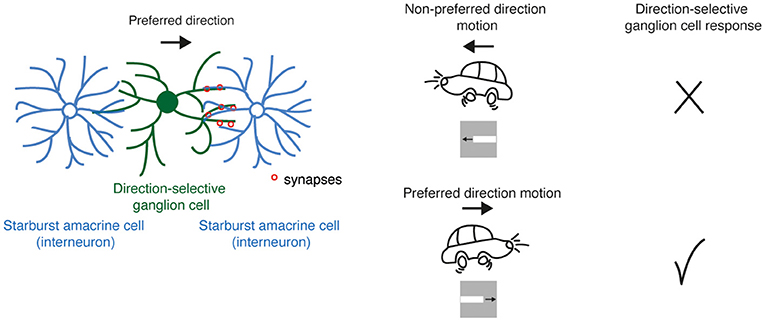
- Figure 2 - The response of direction-selective ganglion cells (green) is determined by the connections it makes with interneurons called starburst amacrine cells (blue), which form synapses (red circles) on only one side of the ganglion cell.
- In the example, the ganglion cell’s preferred direction of movement is to the right. Movement to the left does not cause a reaction in that cell.
This one-sided connectivity allows direction-selective ganglion cells to respond when the movement is in one direction but not when the movement is in the other direction. This is how retinal ganglion cells send complex information to the brain about what the eye is seeing.
The Retina as a Model for the Rest of the Brain
The retina is made of neurons connected by synapses—just like the cells that make up the rest of the brain. As we mentioned earlier, most brain areas have complex connections between neurons that are difficult to study. The retina, too, displays a wealth of precise connections between various cell types, but its organized, layered structure helps researchers understand these connections and how they shape the responses of retinal neurons to visual stimuli. Therefore, the retina is easier to research and understand compared to the rest of the brain. Can we use the retina to help us understand other parts of the brain, despite the retina’s relative simplicity?
Let us take the visual cortex as an example. The visual cortex is part of the cerebral cortex (Figure 1A), which is the outer layer of the cerebrum—the largest part of the brain where the most complex processing takes place. Complex processing can occur due to the cortex’s enormous number of neurons, which connect to each other in convoluted and complicated ways, and which can exhibit various types of responses. Some of these neurons are similar to cells in the retina. For example, in both the retina and the visual cortex, there are cells that respond to light onset or offset, as well as direction-selective cells.
For many years, scientists believed that the visual cortex was very different from the retina, because cells in the visual cortex can adapt and change their responses according to what the animal has seen before. For example, a direction-selective cell in the visual cortex has a certain preferred direction, but if presented with repeated moving stimulation (such as moving white and black stripes—see example in Figure 3A) its preferred direction can be slightly changed and reoriented. It was believed that unlike in the cortex, direction-selective retinal cells could not change their preferred direction. This is because responses in the retina are thought to depend on the connections between direction-selective retinal cells and starburst amacrine cells. So, it was very surprising to discover that cells in the retina can change their responses [2].
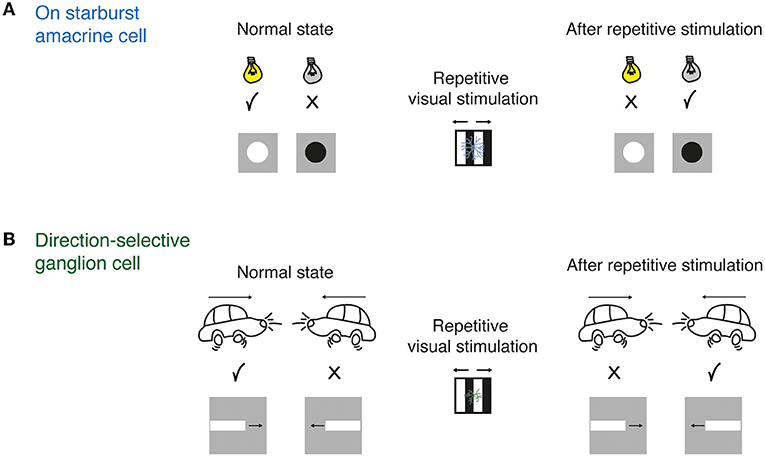
- Figure 3 - (A) The electrical response of a starburst amacrine cell to light and dark.
- Left: the cell reacts to the appearance of light. Right: after exposing the cell to repeating black and white stripes for several minutes, the cell stops responding to light and starts to react to dark. (B) The response of a direction-selective ganglion cell also changes in response to repetitive stimulation. Left: The cell’s preferred direction is to the right. Right: after repeated stimulation, the cell reverses its preferred direction and responds when movement is to the left.
In our research, we exposed the retina to a repetitive visual stimulation (Figure 3A) for several minutes. After a while, On interneurons stopped responding to light onset and started to respond to light offset, like Off interneurons (Figure 3A) [3].
To our surprise, we also found that when direction-selective ganglion cells were exposed to a repetitive stimulus for several minutes, these cells could change their preferred direction (Figure 3B) [4].
Summary
We found that the cells in the retina, like the cells in the cerebral cortex, can change the way they respond to things that are seen. After we found these changes, we asked: what enables cells in the retina to change their responses so dramatically? We used sophisticated techniques that allow us to reveal the inputs to starburst amacrine cells and direction-selective ganglion cells. We found that small differences in the timing of the inputs to the direction-selective ganglion cells can cause dramatic changes in their overall responses and reverse their directional preference [5]. The cerebral cortex is complicated and difficult to study, so learning about the cells of the retina can help us understand how the cells of the cerebral cortex function. What we discovered may help us understand neural mechanisms in other areas of the brain, and could lead to the answer to a central question in brain research: “What happens in our brains when we learn and change?”
Glossary
Synapse: ↑ The connecting site between two neurons, where the transfer of electrical signals takes place.
Retina: ↑ The thin, transparent layer of light-sensitive nerve cells on the back, inner part of the eyeball. The retina converts light into electrical signals that are sent to the visual cortex.
Photoreceptors: ↑ A special type of neuronal cells that can convert light into electrical signals.
Interneurons: ↑ Neurons that transfer information between cells within the same neural network, such as the retina.
Ganglion Cells: ↑ The cells of the retina that send electrical signals about what is being seen from the retina to the brain.
Direction-Selective Ganglion Cells: ↑ A type of ganglion cells in the retina that only respond when there is movement in a certain direction in the visual field.
Starburst Amacrine Cells: ↑ Retinal interneurons that are considered the key players in encoding direction selectivity by asymmetrically connecting to direction-selective ganglion cells on one side.
Visual Cortex: ↑ A part of the cerebral cortex (the outer layer of the mammalian brain) that processes visual information.
Conflict of Interest
The authors declare that the research was conducted in the absence of any commercial or financial relationships that could be construed as a potential conflict of interest.
Acknowledgments
This work was funded by European Research Council (ERC starter No. 757732), I-CORE (grant No. 51/11), and the Israel Science Foundation (1396/15). MR-E is the Sara Lee Schupf Family Chair. LA was supported by ISEF.
References
[1] ↑ Baden. T., Berens, P., Franke, K., Román Rosón, M., Bethge, M., and Euler, T. 2016. The functional diversity of retinal ganglion cells in the mouse. Nature 529:345–50. doi: 10.1038/nature16468
[2] ↑ Rivlin-Etzion, M., Grimes, W. N., and Rieke, F. 2018. Flexible neural hardware supports dynamic computations in retina. Trends Neurosci. 41:224–37. doi: 10.1016/j.tins.2018.01.009
[3] ↑ Vlasits, A. L., Bos, R., Morrie, R. D., Fortuny, C., Flannery, J. G., Feller, M. B., et al. 2014. Visual stimulation switches the polarity of excitatory input to starburst amacrine cells. Neuron 83, 1172–84. doi: 10.1016/j.neuron.2014.07.037
[4] ↑ Rivlin-Etzion, M., Wei, W., and Feller, M. B. 2012. Visual stimulation reverses the directional preference of direction-selective retinal ganglion cells. Neuron 76:518–25. doi: 10.1016/j.neuron.2012.08.041
[5] ↑ Ankri, L., Ezra-Tsur, E., Maimon, S. R., Kaushansky, N., and Rivlin-Etzion, M. 2020. Antagonistic center-surround mechanisms for direction selectivity in the retina. Cell Rep. 31:107608. doi: 10.1016/j.celrep.2020.107608