Abstract
In the field of particle astrophysics, scientists are trying to understand how the universe started and how it works at a very basic level. Using particles from astrophysics sources, we study the laws of physics at the smallest possible scale of matter and develop mathematical formulas that describe how elementary particles interact with each other to make up our universe. My colleagues and I have been studying neutrinos—one of the fundamental building blocks of the universe. That helps us understand how the universe has evolved since it began with the Big Bang some 13.8 billion years ago. In this article, I will tell you about the “ghost particles” called neutrinos—what they are, how we measure them, and why our discovery required a major change in our measurement methods. In this process, you will get to see how the most elusive elements around us are sometimes among the most important.
Prof. Art McDonald won the Nobel Prize in Physics in 2015, jointly with Takaaki Kajita, for the discovery of neutrino oscillations, which shows that neutrinos have mass.
Neutrinos: Fundamental Particles
What is the Universe made of and how has it evolved since the Big Bang. These are several of the most intriguing questions that we can ask ourselves. To answer them scientifically, we can use various approaches and methods. I come from the field of particle astrophysics—a relatively new field of research that studies the basic particles traveling through space, particularly those that reach the Earth. Particle physicists try to understand the basic particles that constitute matter and the forces that govern the interactions between those particles. In general, we try to develop experimental methods to find the smallest particles, called fundamental particles—particles that cannot be subdivided any further. Then, according to what we find, we generate what is called a theoretical model, which is a set of ideas and equations that explains how matter is created from these fundamental particles. We never say that our model is the best one, because each version of a model is based on the level at which we currently understand things, given the sensitivity or resolution of our current instruments. Over the years, instruments become progressively more sensitive and, consequently, we learn new and exciting things about the fundamental building blocks of matter and the universe we live in.
The long-standing model describing particles and the forces between them is called the standard model [1]. (To learn more about the standard model, see here.) According to the standard model, all matter, including the atoms that build our bodies, the air we breathe, and the light we receive from the Sun, is composed of fundamental particles. These particles were created during the big bang, some 13.8 billion years ago, and during subsequent evolution of the Universe.
Fundamental particles include electrons, quarks, and neutrinos (Figure 1A), as well as other particles you might have heard of, like photons, bosons, gluons, and Higgs particles. We will focus on neutrinos in this article. All the fundamental particles interact with each other through four fundamental forces, called the strong force, the weak force, the electromagnetic force, and the gravitational force. Quarks are the building blocks of both protons and neutrons. Neutrons and protons make up the nuclei of atoms, which are surrounded by electrons (Figure 1B).
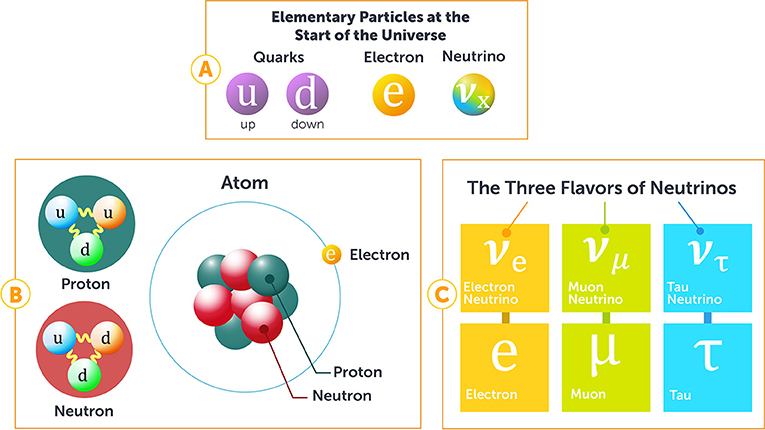
- Figure 1 - Elementary particles.
- (A) According to the standard model, the universe began with the formation of elementary particles called quarks, electrons, and neutrinos. There are several types of quarks, among them are up (u) quarks and down (d) quarks, and three types of neutrinos (νx, where ν designates neutrino and x stands for one of the three types). (B) Quarks are the building blocks of both protons and neutrons. Neutrons and protons make up the nuclei of atoms, and electrons circle around atomic nuclei. (C) Neutrinos come in three types, or flavors, and they interact with three elementary particles: the electron, the muon, and the tau.
Neutrinos are emitted by substances that are naturally radioactive, and during certain reactions that we can create in scientific devices called accelerators. However, neutrinos are most commonly generated by nuclear reactions in the Sun, in a process called nuclear fusion. In nuclear fusion, two atomic nuclei combine to form a single, heavier atom while releasing massive amounts of energy and particles including neutrinos. These neutrinos take 2 s to exit the sun and about 8 min to reach Earth. Their number is phenomenal—to give you an idea, every second, each square centimeter of the Earth’s surface is crossed by 65 billion solar neutrinos!
Neutrinos are unusual elementary particles because they interact with matter by only two of the four fundamental forces—gravity and the weak force (the weak force can enable a neutrino to change a neutron into a proton and an electron). Since neutrinos are nearly massless, the force of gravity they exert is extremely small and practically undetectable. As for the weak force, they must come extremely close to other protons, neutrons, or electrons to interact with them. This makes neutrinos extremely hard to detect [2]. Neutrinos can basically pass through ordinary matter as though it were almost transparent. In fact, neutrinos interact with matter only when they hit the nucleus of an atom or the electrons revolving around a nucleus head-on, and this happens quite rarely because atoms are mostly empty space. In all other cases, neutrinos pass through matter uninterrupted—including many billions of them that pass through our bodies every second! Because neutrinos only interact very weakly with our detectors, it is extremely difficult to see them and measure their properties. Due to their rare interactions with matter, some people call neutrinos “the ghosts of the universe.”
Although elusive and hard to measure, neutrinos play a central role in the formation of the universe. They help to build structures like stars and galaxies. They also helped to generate some of the basic elements created at the start of the universe during the Big Bang.
Neutrinos come in three types, or flavors, called the electron neutrino, muon neutrino, and tau neutrino. Each flavor interacts with the corresponding elementary particle—electron, muon, and tau (Figure 1C) [3]. We do not know exactly why there are only three types of neutrinos, but these are the types we have found so far and they fit the predictions of the standard model. As you will see below, our important discovery, for which I was awarded the Nobel Prize in Physics in 2015 jointly with Prof. Takaaki Kajita, is related to changes in the flavors of neutrinos as they travel through space, from the core of the Sun to the Earth.
How We Measured Neutrinos
When we started our research on neutrinos, there was an unresolved problem in particle astrophysics called the solar neutrino problem [4]. To measure neutrinos, special detectors had been built, but these detectors showed that the number of observed electron neutrinos coming from the Sun was much lower than the expected number, based on very solid calculations of how the Sun burns. This discrepancy between the measured and expected number of neutrinos arriving from the Sun to the Earth meant one of two things: either we needed to update the standard model of elementary particles and alter the way we think about neutrinos, or we needed to change the way we calculate the number of neutrinos arriving from the Sun. Both possibilities had significant implications for our understanding of the universe and, therefore, many particle astrophysicists were on a collective mission to design an experiment that could solve the solar neutrino problem.
As mentioned previously, neutrinos cannot be measured by direct interaction with our detectors. Instead, neutrinos are normally measured indirectly, using effects that take place when fundamental particles are emitted in radioactive processes. For example, an electron neutrino can be measured using a radioactive process called beta decay, during which an electron is emitted. We can measure the energy of the emitted electrons, and scientists originally believed that only electrons are emitted in this process, so they expect to measure a single energy for all electrons emitted. Instead, they get a whole range of lower energies from the emitted electrons! To account for this range of energy release, they assumed that another particle (the electron neutrino) was also released. In this way, they indirectly measured electron neutrinos through the “missing energy” of electrons emitted during beta decay.
In our experiment at the Sudbury Neutrino Observatory (SNO), 2 km deep in the ground in Canada (Figure 2A and Appendix), we used a similar approach to indirectly measure neutrinos through their effect on a special type of water called heavy water. As you know, regular water (H2O) is comprised of one atom of oxygen (O) and two atoms of hydrogen (H). Hydrogen has one proton in its nucleus. In contrast, heavy water (D2O) contains one atom of oxygen but two atoms of deuterium (D). Deuterium has one proton and one neutron in its nucleus (in other words, it is a hydrogen atom with an additional neutron). This increases its weight by 10% but does not change its chemical properties very much. Heavy water occurs naturally, such that 1 in every 6,400 molecules of water is D2O.
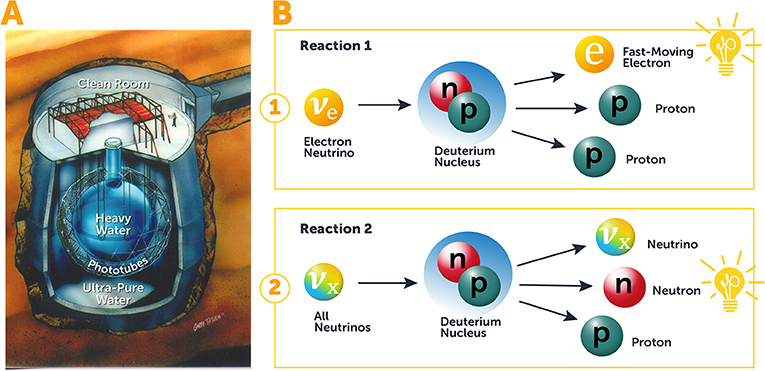
- Figure 2 - The underground Sudbury Neutrino Observatory for detecting neutrinos.
- (A) The SNO neutrino experiment was performed some 2,100 meters underground. It was designed to detect solar neutrinos through their interactions with heavy water. The observatory included a clean room from which scientists lower equipment into the measurement area, and a measurement area filled with ultra-pure water, to block radioactivity coming from the surrounding rock. The acrylic sphere in the center was filled with heavy water and surrounded by a sphere containing phototubes to measure the effects of neutrinos hitting the heavy water (Image credit: Prof. McDonald). (B) We measured two reactions: (1) Interactions of electron neutrinos with the deuterium nucleus, and (2) interactions of all three favors of neutrinos with the deuterium nucleus.
In our SNO experiment, we filled a big container with pure heavy water and measured the effects of collisions between neutrinos arriving from the Sun with that heavy water. Basically, we measured two reactions that occur when neutrinos collide with heavy water. In the first reaction, an electron neutrino interacts with a deuterium atom of the heavy water. This interaction changes the neutron in the atom’s nucleus into a proton and a fast-moving electron that produces light (Figure 2B, Reaction 1), and we measured the light that this electron produces. In the second reaction, neutrinos of all three flavors (electron, muon, and tau) interact with a deuterium atom. In this interaction, the nucleus of the deuterium atom breaks into a proton and a free moving neutron. The free neutron moves through the heavy water and is detected in different ways in the three phases of the project. In the first phase, the neutron is captured by another deuterium atom, producing light with different properties than reaction 1 (Figure 2B, Reaction 2).
So, we had two light-producing reactions of neutrinos with heavy water that we could measure using our light sensors, called phototubes—therefore, we could indirectly measure the presence of neutrinos.
It took a great deal of effort to make sure that we were measuring only the effects of neutrinos and no other sources of radiation. We had to shield our detectors from the radioactivity coming from outer space, which is why we had to place the detectors some 2 kilometers underground, surrounded by rock (Figure 2A). We also had to make sure that we were not measuring radioactivity coming from the rock itself. Specifically, we had to shield our heavy water area from uranium and thorium—two radioactive elements present in rocks. To do so, we surrounded our heavy water container with ultra-clean water—billion of times cleaner, in terms of radioactive elements, than tap water. This clean water captured the products of radioactivity from the rock. We also built the detector from materials carefully selected to be low in radioactivity and created ultra-clean air and ultra-clean workers who took showers and wore lint-free clothing.
To measure the light emitted when the neutrinos interacted with the heavy water, we placed many phototubes around the heavy water container. Creating this experimental setup was very challenging—it was both a major engineering task and a complex physics experiment. (To learn more about the engineering aspect of the project, see the Appendix.)
Where are the Missing Neutrinos?
As mentioned above, our challenge was to solve the solar neutrino problem, whereby the number of electron neutrinos measured as reaching Earth was about three times smaller than the expected number. Either the experiment or the theory (or both) could have been incorrect, or maybe the electron neutrinos from the sun were changing flavors and escaping detection in experiments that were solely or primarily sensitive to electron neutrinos.
In our experiment, we wanted to check whether flavor-changing was happening before the neutrinos reach the Earth. We knew that, in the core of the Sun, only electron neutrinos are produced (muon and tau particles are heavier than electrons and therefore producing them and their associated neutrinos requires more energy than is available in the Sun). This means that, if some of the neutrinos arriving from the Sun are not electron neutrinos, they must have changed flavor while traveling from the core of the Sun to the Earth. (Neutrinos change flavors in a periodic manner, through a quantum phenomenon called neutrino oscillations. You can read more about it here.) By tuning our detectors to a specific range of energy, we could detect the effects of neutrinos originating from the Sun, and not from other sources like the cosmic rays that emit neutrinos with higher energies. At the energies we studied, the Sun is by far the main producer of neutrinos reaching the earth.
In one measurement with our detector we observed electron neutrinos interacting with deuterium atoms and emitting a fast-moving, free electron, as described above. In a separate measurement, we observed neutrinos of all three flavors interacting with deuterium atoms and emitting a free-moving neutron. In other words, the first measurement told us how many electron neutrinos arrive from the Sun, while the second measurement told us the total number of all neutrinos coming from the Sun. Comparing the two, we found that only one-third of the total neutrinos arriving from the Sun are electron neutrinos. Therefore, two-thirds of the neutrinos changed their flavor from electron neutrino to either muon neutrino or tau neutrino (Figure 3) [2, 5]. Our experiment showed that an electron neutrino can change flavors as it travels—this was the solution to the solar neutrino problem!
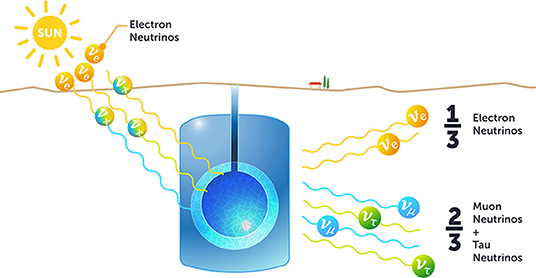
- Figure 3 - Neutrinos change flavors as they move from the core of the Sun to Earth.
As part of the standard model for elementary particles, it was originally assumed that neutrinos have no mass and that they travel at the speed of light. The discovery that neutrinos oscillate implied—according to considerations resulting from Einstein’s theory of relativity—that neutrinos do have mass. It is beyond the scope of this paper to explain in detail why the fact that neutrinos change flavor through space means that they have mass. But, in general, Einstein’s theory of special relativity determines that this periodic change in flavor means that, from the point of view of neutrinos, time is passing. An experience of time implies that neutrinos move slower than the speed of light, and therefore have mass. Our experiment, along with measurements made at the Super-Kamiokande experiment in Japan with whom we shared the Nobel Prize, provided the first evidence for physics that goes beyond the standard model. The extension of the standard model will give us a more complete understanding of our universe at a very basic level. A large number of people worked for an extended period of time to make this great achievement possible. I am deeply grateful to everyone involved in this important project and I feel fortunate to have participated. Through being awarded the Nobel Prize, I see myself as representing all my highly skilled, dedicated colleagues who made this project successful.
Recommendation for Young Minds
I grew up in a very small, steel-manufacturing city in Canada. While people there appreciated the value of education, no one expected a resident to end up winning a Nobel Prize. This means that any one of you—if you work hard enough and find really good people to work with—can doing something really significant with your life, and perhaps win a prize like the Nobel Prize.
When it comes to choosing your career, I say pick a few things that you will be happy to do when you wake up in the morning, and then try them out. Then, see which ones you are good at—that is what I did! I believe it is a very good way to choose your career. After you choose something, just keep working at that career, and maintain positive, friendly relationships with the people around you—they are highly important for your success.
It is also very important to remain curious throughout your life, as the world as a whole, and science in particular, keep changing quickly. You might not believe it, but when I was in university in 1964, the university received its first computer. It was so large and heavy that it had to be lifted with a crane and lowered into the physics building through its roof! Nowadays, many of you probably have portable computers or even cell phones that are much more powerful and vastly smaller than that those early computers (Figure 4). This is one example of how much science has changed during my career, and I think this amazing pace will continue. Therefore, keep being curious, learn new things, and adapt to new advancements. Furthermore, remember that it is you, the young people, who are best at working with and developing new technologies—so you have a lot to contribute! Therefore, do not hesitate to learn as much as you can about the latest technologies, and try to pass that knowledge on and educate others—even your older colleagues.
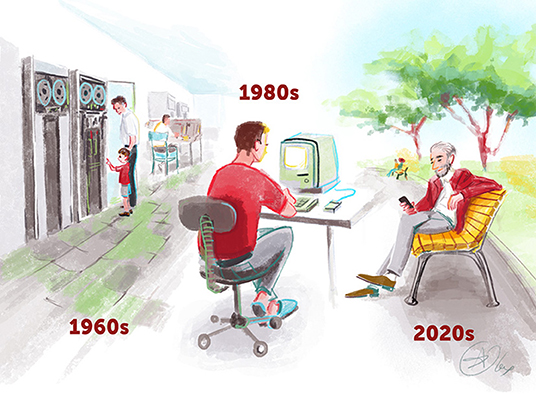
- Figure 4 - Keep curious, as the world is changing rapidly.
- Technology has advanced rapidly since I was a student in the 1960s, and I believe this fast pace will continue into the future.
Additional Materials
Ain’t no stopping them now with Art McDonald (Nature video).
Glossary
Fundamental Particles: ↑ The smallest particles that make up all other particles.
Standard Model of Fundamental Particles: ↑ A model for the fundamental particles and their interactions through the forces of nature.
Neutrinos: ↑ Fundamental particles that interact by gravity and the weak force.
Radioactivity: ↑ A spontaneous emission of energetic particles resulting from the breakdown of atomic nuclei.
Neutrino Flavor: ↑ A characteristic of neutrinos that defines their type. Neutrinos come in three distinct flavors—electron neutrino, muon neutrino, and tau neutrino.
Heavy Water: ↑ Water containing deuterium atoms instead of hydrogen atoms. Deuterium has one proton and one neutron in its nucleus, whereas hydrogen atom has only a proton. It behaves chemically like hydrogen.
Phototubes: ↑ Light sensors that help us measure the light produced when neutrons interact with heavy water.
Conflict of Interest
The author declares that the research was conducted in the absence of any commercial or financial relationships that could be construed as a potential conflict of interest.
Acknowledgments
I wish to thank Noa Segev for conducting the interview which served as the basis for this paper, and for co-authoring the paper, and Alex Bernstein for providing Figures 1–4.
References
[1] ↑ Cottingham, W. N., and Greenwood, D. A. 2007. An Introduction to the Standard Model of Particle Physics. New York, NY: Cambridge University Press.
[2] ↑ McDonald, A. B. 2016. Nobel lecture: the Sudbury Neutrino Observatory: observation of flavor change for solar neutrinos. Rev. Modern Phys. 88:030502. doi: 10.1103/RevModPhys.88.030502
[3] ↑ Acker, A., and Pakvasa, S. 1997. Three neutrino flavors are enough. Phys. Lett. B. 397:209–15. doi: 10.1016/S0370-2693(97)00174-3
[4] ↑ Haxton, W. C. 1995. The solar neutrino problem. Annu. Rev. Astron. Astrophys. 33:459–503.
[5] ↑ Ahmad, Q. R., Allen, R. C., Andersen, T. C., Anglin, J. D., Barton, J. C., Beier, E. W., et al. 2002. Direct evidence for neutrino flavor transformation from neutral-current interactions in the Sudbury Neutrino Observatory. Phys. Rev. Lett. 89:011301. doi: 10.1103/PhysRevLett.89.011301
Appendix
Overview: The Sudbury Neutrino Observatory Experiment
The SNO experiment for measuring neutrinos and their flavors was a great collaborative effort. At any given moment, more than 150 people were working on the experiment, each responsible for a certain part. First, we had to excavate an enormous cavity, 2 km underground, in a former mine in Sudbury, Canada. The construction team had to drill holes in the floor of the cavern and place explosives there. They then had to lift all their equipment out of the cavity and set off the explosives to deepen and widen the cavity. After that, they had to remove the rubble created by the explosion. It took about 2.5 years and 8 sets of explosions to create this 34-meter high (the height of a 10-story building), 22-meter-wide cavity.
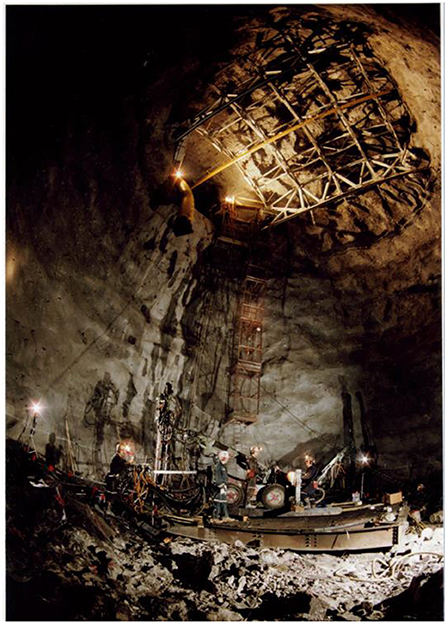
- Appendix Figure 1 - The construction team placing explosives to create the cavity for the SNO.
After creating the cavity, we had to build the acrylic sphere that would contain the heavy water. The sphere was constructed out of 120 pieces, each small enough that it could be lowered into the mine using the elevator.
Next, we had to construct a geodesic sphere around the acrylic sphere, where the photosensors would be placed to measure the effects of neutrinos reacting with heavy water. Overall, we installed 10,000 photosensors on the geodesic sphere, using lifts.
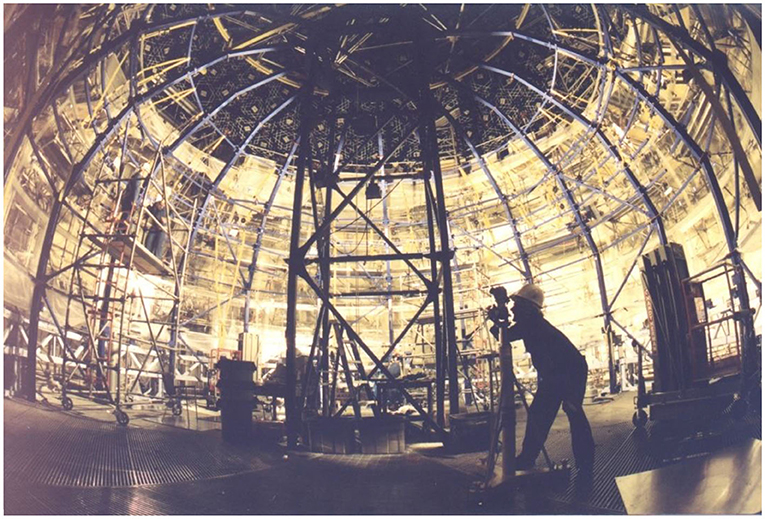
- Appendix Figure 2 - Placement of the top 60 (out of 120) pieces of the acrylic sphere that would contain the heavy water.
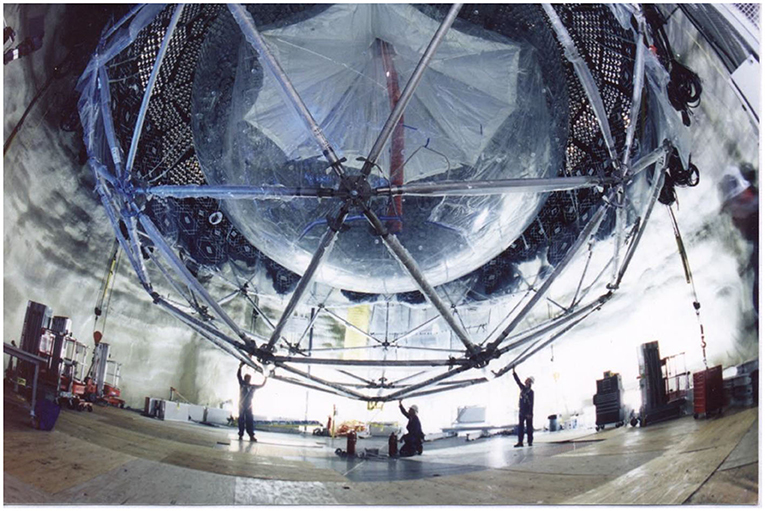
- Appendix Figure 3 - Construction of the geodesic dome around the acrylic sphere, before the installation of photosensors to detect the neutrinos.
Eventually, we filled up the acrylic sphere with 1,000 tons of pure heavy water (D2O). The water was so pure that we had less than one spontaneous radioactive decay per day per ton of water, which is a billion times purer than tap water. Even with such a huge amount of pure heavy water, we could measure the effect of only 1 neutrino arriving from the Sun per hour, because neutrinos rarely interact with matter.
As you can see, this project was both a complex engineering task and a fundamental physics experiment. Many skilled, dedicated people worked in collaboration toward a common goal that they felt was significant. We often had to choose how to go about the experiment and we did so by communicating with each other in detail about the alternatives, until it was clear that the group favored one alternative over the other. Fortunately, we were able to reach agreement after having good discussions. By working together in this collegial way, we made our experiment successful and, consequently, we learned something new and significant about the fundamental building blocks of our universe.
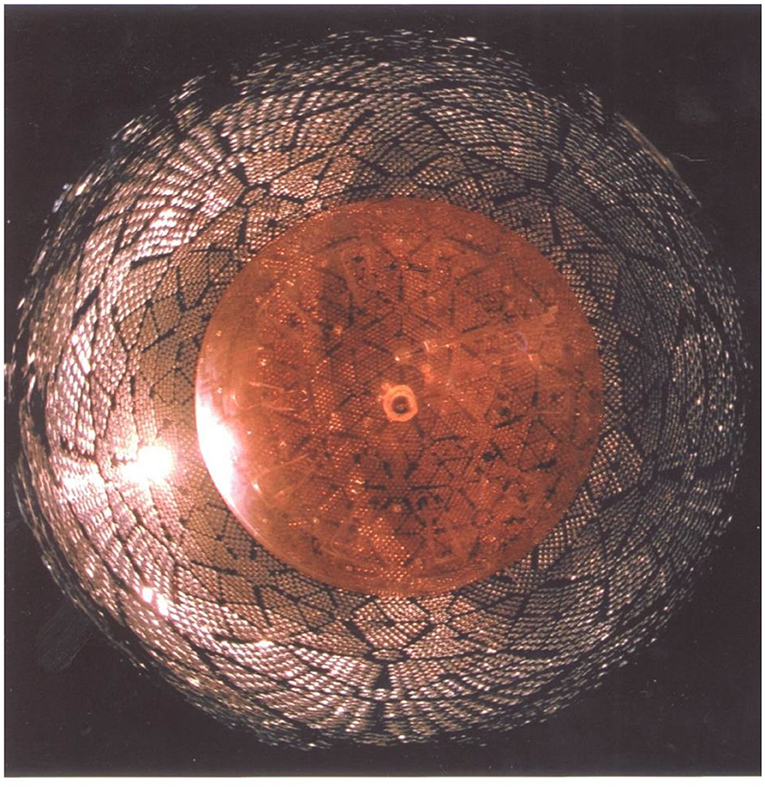
- Appendix Figure 4 - A wide-angle shot looking up at the bottom of the acrylic sphere (red), surrounded by 10,000 photosensors used to detect the presence of neutrinos.