Abstract
Earth’s atmosphere and natural magnetic field do a good job of protecting us from space radiation. Space radiation is different from radiation on Earth, which mostly comes from sources found in rocks and soil or from medical procedures like X-rays. Space radiation comes from particles ejected from the Sun, or from the explosions of dying stars (called supernovae) outside our solar system. These particles have been stripped of their electrons as they are accelerated in space to almost the speed of light. One of NASA’s biggest challenges is protecting astronauts from these high-energy particles, called galactic cosmic rays (GCRs), because they can cause cancer and other diseases. To understand the biological damage caused by GCRs and to develop ways to protect astronauts, NASA has built a galactic cosmic ray simulator on Earth.
What is Galactic Cosmic Radiation?
Galactic cosmic rays (GCRs) are the main radiation health hazard for humans traveling on long missions through space. GCRs come from outside our solar system and are likely formed by supernovae—major explosions that happen when a star reaches the end of its life. While radiation sources on Earth generally include things like gamma radiation and X-rays (like the ones you get at the doctor or dentist), GCRs consist of the nuclei of the elements in the periodic table, including hydrogen through uranium. When all of an atom’s electrons have been stripped off, they are called fully ionized particles. GCRs move extremely fast—some of the particles approach the speed of light. Space radiation is called mixed-field radiation, which means it contains a variety of particles, all moving at different speeds. These energetic particles come from all directions in space, and they are much more damaging to living things compared to the types of radiation found on Earth (Figure 1). Understanding this difference is fundamental to estimating health risks faced by our astronauts [1].
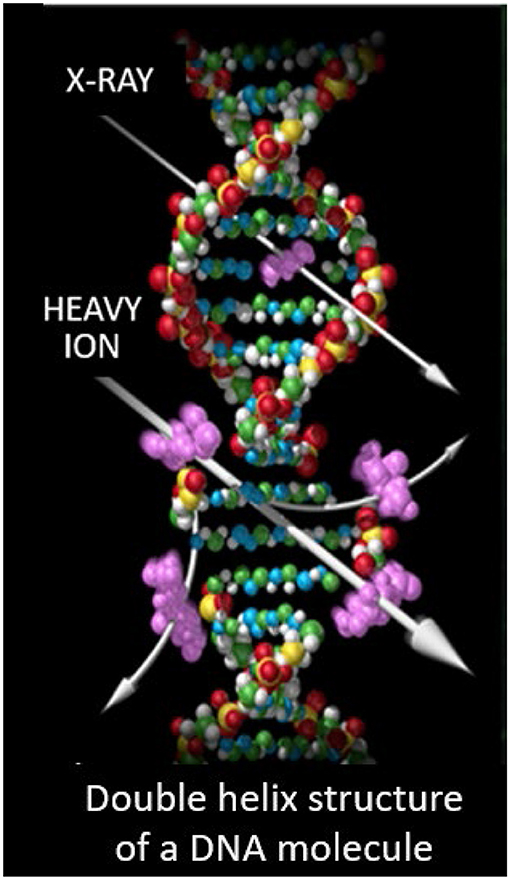
- Figure 1 - Galactic cosmic rays contain heavy ions that can pass through human cells and cause DNA damage (purple) that is much more difficult for the body to repair compared to radiation found on Earth, such as x-rays or gamma radiation (image courtesy of NASA).
Hydrogen alone accounts for ~87% of the GCR particles; helium accounts for ~12%, and all particles heavier than helium (called heavy ions) account for the remaining 1% [2]. Although there are far fewer heavy ions compared to hydrogen and helium, they are very damaging to living things, and estimating the health risk they present is challenging. There is a broad range of energies associated with each of the particles found in GCRs. For lower-energy GCRs, a few centimeters of living tissue or spacecraft shielding can fully stop the particles. For higher-energy GCRs, many meters of shielding still cannot fully stop the particles—these particles are moving at nearly the speed of light! Scientists are focused on testing radiation damage across this entire energy range.
To make things even more complex, GCRs can be changed as they interact with spacecraft shielding and human tissues. These interactions can slow down GCR particles and cause the production of new particles, which are referred to as secondary radiation. The secondary radiation includes atomic particles such as neutrons, which can penetrate further into living things and cause even more damage than the incoming GCR. The radiation environment encountered by astronauts inside their spacecraft is therefore a combination of primary GCR particles that penetrate through shielding and secondary radiation produced by the interactions of GCR with matter.
How much Radiation Exposure does an Astronaut Receive?
An astronaut’s daily exposure to GCRs not only depends on the amount of shielding provided by their spacecraft, but also the length of the mission and where they are in our solar system. Close to home, Earth’s protective magnetic field can provide a significant amount of protection to astronauts on the International Space Station (ISS), for example. For missions to the moon and Mars, the largest exposures happen further out in space. Once on the surface, the mass of the moon or planet will protect astronauts from half of the GCRs. On Mars, the atmosphere provides some additional protection. In addition to the shielding provided by the spacecraft, the tissues of the human body itself provide some protection to critical organs.
To estimate astronaut radiation exposures, NASA uses a combination of tools and measurements to calculate the amount of radiation reaching sensitive organs such as the lungs, heart, and brain. Next, an estimate of the amount of biological damage can be calculated based on the dose and type of radiation received. Biological damage is described using a unit called Sieverts (Sv). Astronaut exposures range from ~20 mSv (“milli”Sv, or 10-3 Sv) for short space shuttle-type missions to over 300 mSv for long stays on the ISS. Astronauts on a short 30-day lunar mission will receive ~40 mSv, while exposures for a Mars missions, which would last nearly 3 years, are on the order of 1,200 mSv. Compared to workers on Earth, with average exposures of <3 mSv per year, astronauts receive the most radiation of any modern radiation worker.
What are the Radiation Health Risks to Astronauts for Long-Duration Missions?
Astronauts face risks from exposure to GCRs both while they are on space missions and long after their missions are complete. During missions with high exposures like a Mars mission, the major risks are possible changes in the brain, resulting in poorer performance during flight. After spaceflight, astronauts face increased risks of blood cancers (e.g., leukemias), solid cancers (e.g., lung or gastrointestinal tumors), heart-related diseases, and the potential for worsening brain-related problems later in life (for more details about the health risks faced by astronauts, see this Frontiers for Young Minds article). NASA is working hard to increase understanding of both in-flight and long-term health risks, so that they can better protect astronauts from them.
How can We Simulate Space Radiation on Earth?
One way to learn about the radiation threats astronauts face is to study them here on Earth. But, as we mentioned earlier, the radiation on Earth is different from the radiation in space. So first, we must find a way to reproduce space-like radiation on Earth. Large particle accelerator facilities are needed to speed up particles to energies high enough to mimic the space environment. However, these facilities can only produce one type of particles at a time—and, as we mentioned, space radiation is a mixed-field of radiation containing essentially all of the elements in the Periodic Table! This makes mimicking space radiation on Earth quite a challenge.
In 2003, NASA began using the NASA Space Radiation Laboratory (NSRL) at Brookhaven National Laboratory to perform its ground-based, heavy-ion research (Figure 2). Here and around the world, most research on understanding health risks from exposure to space radiation has been performed using exposures to one type of particle at one energy. Over the last decade, upgrades made to the NSRL have helped the facility to more closely approximate the radiation exposures found in space. Today, the facility can supply many types of particles with a variety of energies during a single radiation experiment by switching from one type of particle to another every 2–4 min [3]. While particle energies are still much lower than the GCR field outside the spacecraft, the NSRL can simulate the range of GCR particles and energies encountered by astronauts while inside their spacecraft, which have slowed down considerably while moving through the spacecraft’s shielding.
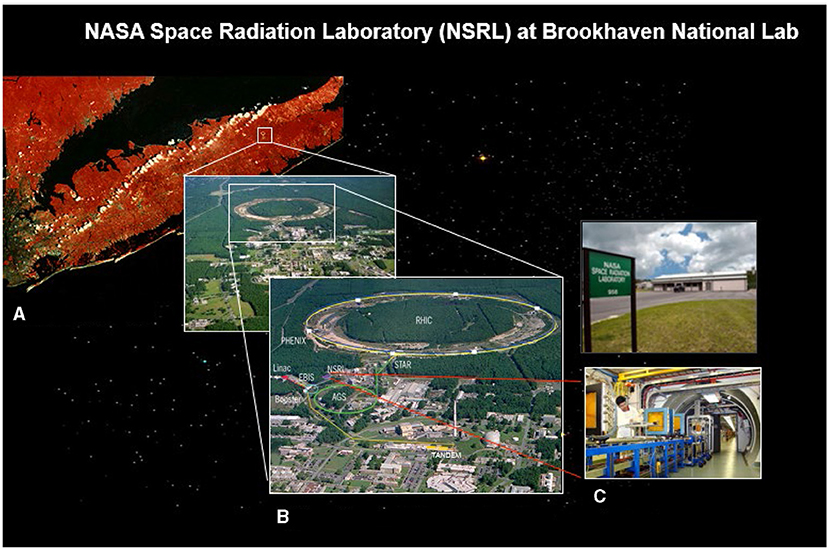
- Figure 2 - (A) Satellite image of Long Island, New York, where Brookhaven National Laboratory is located.
- (B) Aerial photo showing the large accelerator facility needed to create high-energy ions. For scale, the top “RHIC” ring is 2.4 miles in circumference. NSRL uses a “booster” ring, which is ~286 feet in circumference and lined with powerful magnets to accelerate ions. (C) Inside the NSRL target room where experiments are performed (images courtesy of BNL).
NSRL is the only facility that can closely simulate space radiation. NSRL systems and controls software have been optimized to deliver beams of particles reliably and rapidly (Figure 3). Each GCR simulation utilizes 21 switches of unique energy-ion beam combinations. A typical exposure requires ~75 min to deliver. To simulate lower dose rates more closely, several smaller exposures can be given daily over 2–6 weeks. Simulating the low GCR dose rates found in space remains one of NASA’s greatest challenges.
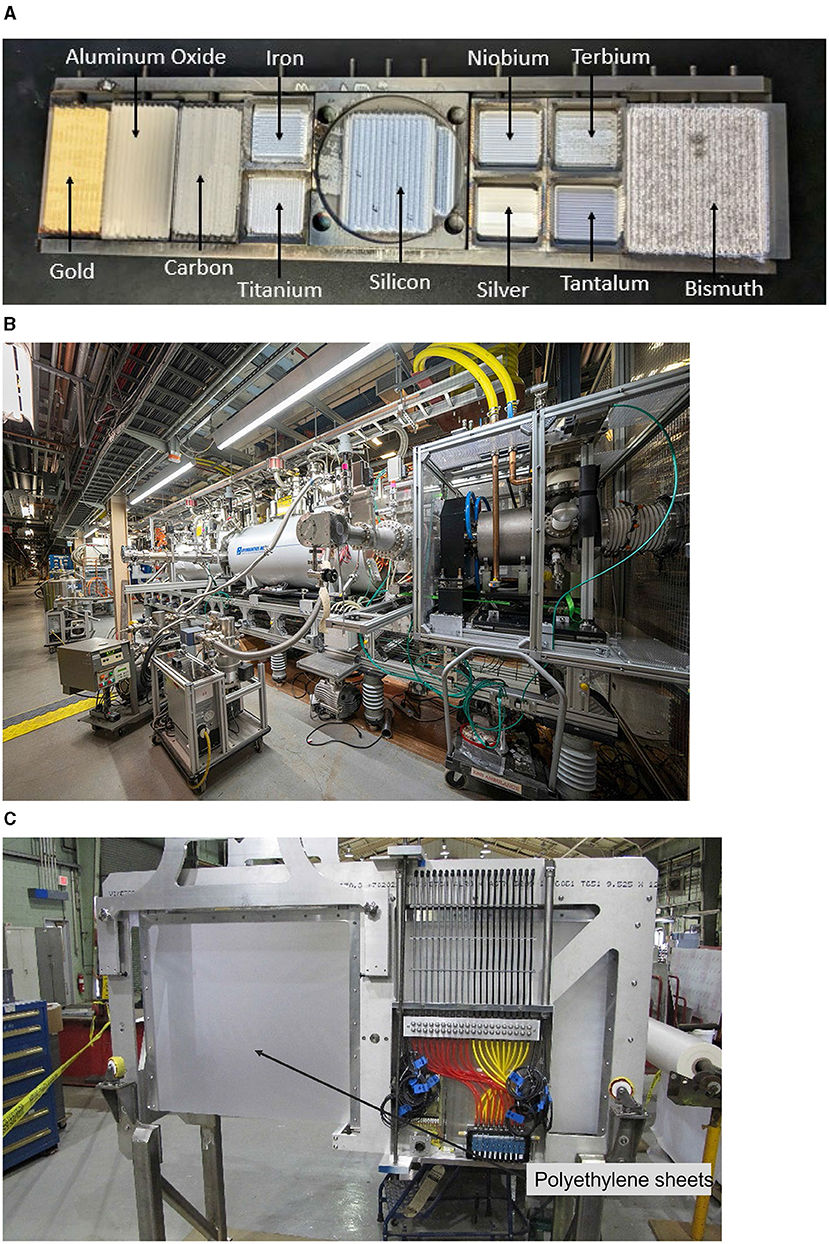
- Figure 3 - Complex systems and machinery create and accelerate ions from solid and gas sources.
- (A) A laser is used to evaporate high-purity solid targets to produce ions needed for the simulation. The target holder is 25 cm by 6 cm. The iron target is roughly the size of a US quarter. (B) The Electron Beam Ion Source further strips these ions of their electrons before delivery to the “booster ring” which accelerates ion beams to high energies. (C) To produce the lowest energy beams, some ions are slowed down by inserting sheets of polyethylene into the beamline (images courtesey of BNL).
How is the Simulator Being Used by NASA?
NASA is using the GCR simulator to develop protection strategies to reduce impacts to astronauts’ health and to keep astronauts performing at their best on future long-duration missions. While we have learned a lot from astronauts living on the ISS, many details remain unknown about the radiation health risks faced by astronauts—especially from the large radiation exposures that will be experienced during a Mars mission. The GCR simulator provides researchers the ability to conduct mixed-field radiation studies on Earth, under controlled conditions. Research teams are using cells and experimental animal systems, as well as advanced tissues-on-a-chip technologies, to study the effects of mixed-field radiation. NASA will use these results to better estimate radiation health risks and to update radiation exposure limits if needed. The GCR simulator also allows scientists to test the methods that could be used to protect our astronauts. Multiple research teams are currently testing the effectiveness of a variety of medicines and dietary supplements, such as antioxidants and anti-inflammatory drugs, to reduce radiation damage to sensitive tissues.
What Key Simulation Challenges Remain?
Scientists still have major questions to answer about space radiation. Key challenges remain in determining whether the GCR simulator mimics the radiation environment in space closely enough to accurately predict health effects for humans. Scientists are still learning how to run these tests properly and are working to better simulate deep-space radiation exposures, including figuring out which animals and cell types provide the best information for how humans respond to space radiation. Research results are expected soon and will help NASA to protect the brave individuals doing the important and exciting job of exploring space.
Glossary
Galactic Cosmic Rays: ↑ High energy particles, including protons and heavy ions, that move through space at nearly the speed of light.
Gamma Radiation: ↑ A high energy form of electromagnetic radiation with a short wavelength arising from the radioactive decay of atomic nuclei.
Fully Ionized Particles: ↑ Atoms that have been stripped of all their electrons.
Heavy Ions: ↑ Nuclei of the elements with charge number >3 such as oxygen, carbon, or iron that are positively charged because some or all (fully ionized) of their planetary electrons have been stripped.
Secondary Radiation: ↑ Radiation, including neutrons, produced by interaction between the primary space radiation and matter such as shielding.
Sievert (Sv): ↑ Unit of measure to reflect biological damage from a given dose of radiation.
Particle Accelerator: ↑ Is a machine that uses electromagnetic fields to propel charged particles to very high speeds and energies.
Antioxidants: ↑ Man-made or natural substances that may prevent or delay some types of cell damage caused by free radicals or unstable molecules.
Conflict of Interest
The authors declare that the research was conducted in the absence of any commercial or financial relationships that could be construed as a potential conflict of interest.
Original Source Article
↑Simonsen, L. C., Slaba, T. C., Guida, P., and Rusek, A. 2020. NASA’s first ground-based Galactic Cosmic Ray Simulator: enabling a new era in space radiobiology research. PLoS Biol. 18:e3000669. doi: 10.1371/journal.pbio.3000669
References
[1] ↑ Cucinotta, F. A., and Durante, M. 2006. Cancer risk from exposure to galactic cosmic rays: implications for space exploration by human beings. Lancet Oncol. 7:431–5. doi: 10.1016/S1470-2045(06)70695-7
[2] ↑ Slaba, T. C., and Whitman, K. 2020. The Badhwar-O’Neill 2020 model. Space Weather 18:e2020SW002456. doi: 10.1029/2020SW002456
[3] ↑ La Tessa, C., Sivertz, M., Chiang, I. H., Lowenstein, D., and Rusek, A. 2016. Overview of the NASA space radiation laboratory. Life Sci. Space Res. 11:18–23. doi: 10.1016/j.lssr.2016.10.002