Abstract
What is sound? How does it move? What exactly are the characteristics of sound, like amplitude and pitch? In this introduction to the World of Sound, we present several simple experiments you can do at home, that can help young readers like yourself to find the answers. Find a coat hanger, a slinky, a flowerpot, and your favorite grown-up assistant...and get started!
Sound Is All Around!
Sound is all around us. From the crunching of leaves underfoot on an autumn walk, to the click-clack of a keyboard when we type, we make sounds just by being alive! Often, sounds are useful: we use our voices to communicate, make music, and laugh. An alarm alerts us to danger, and we listen for traffic when crossing the road. But sounds can be unhelpful, too: noise can damage our hearing and disrupt our sleep. Understanding sound can help scientists and engineers make use of the good aspects of sound and minimize the bad. In this article, we will conduct some fun experiments that explore the concept of sound. We will discover what sound really is, find out how it travels, and learn what makes one sound different from another. We will do all of this using everyday objects! So, grab your adult helper and we can get started!
What Is Sound?
You may have heard that sound is a vibration, but what exactly does that mean? Gently put two fingers on your throat and hum loudly. Can you feel movement? That is your voice box vibrating! But how does the sound get from your vibrating voice box to a listener’s ears?
When you hum, speak, or sing, you are actually forcing air through your voice box. This causes the insides of your voice box to vibrate. These vibrations cause the skin, tissues, and air inside your breathing tubes to vibrate too, and the sound spreads like the ripples on a lake. If you are speaking or singing, the vibrating air comes out through your open mouth—and, if you are humming, probably from your nose—but in both cases, the vibrating skin of your throat causes air molecules touching the skin to vibrate, too (see also below). These vibrations spread outward from your body, through everything around you.
Eventually, some of these vibrations will reach someone’s ears. They then travel down long tubes in each ear, called the ear canal, and hit the listener’s ear drums—thin pieces of skin stretched over the ends of the ear canals. The ear drums also start to vibrate, and this sends signals to the listener’s brain, which then recognizes these vibrations as sound.
Sound Travels in Waves
Although sound needs a substance like air to travel through, the air itself does not travel—it is the vibrational movement that travels from molecule to molecule. We call this a sound wave.
Try this:
- Hold a slinky on a flat surface like a table top, with one end in each hand.
- Pull your hands as far apart as you can, so the slinky is stretched.
- Now, move your right hand back and forth toward the left, keeping your left hand still.
What happened? If you watched carefully, you probably saw the slinky coils bunch up, and it probably looked like the bunch of coils was moving toward your left hand. The coils of the slinky are like the air molecules in a sound wave. They first bunch together (this is called a compression), and then move apart (this is called a rarefaction). It might look like the bunched-up coils are traveling along the slinky, but if you mark one coil with a piece of sticky tape, you will see that the bunched coils are actually moving back and forth around a fixed point. Another way to show how waves travel is a jelly-bean wave machine, here demonstrated by the UK National STEM Centre.
Crowds of Molecules
Now let us look at the movement of sound in terms of molecules. You know that substances like air, water, wood, and stone are made up of trillions of miniscule particles called molecules. Molecules in solids are closer together than molecules in the air. All these molecules crowded together behave like a crowd of people. Imagine you are in the middle of that crowd, jostling for space with the people around you. If you try to move, you will probably push against the other people around you, causing them to move too. The tighter the crowd, the more one person’s movement affects the others.
Sounds travels through substances in a similar way. Because the molecules in liquids and solids are closer together than those in the air, sound moves faster through these substances than it does through the air. In fact, sound travels through the air at about 340 meters per second, whereas it travels through water at close to 1,500 meters per second. That is the same as running almost four times around a running track in just ONE SECOND! Let us try another experiment to learn more (Figure 1).
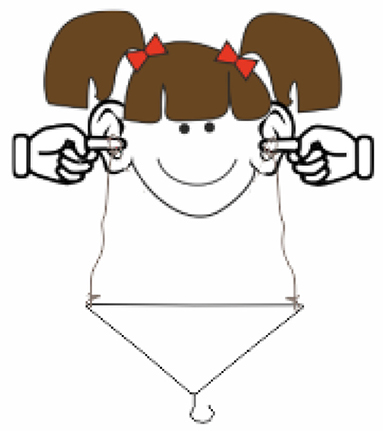
- Figure 1 - Two strings and a coat hanger to demonstrate the different sound speed in air and in solids.
- When you put the fingers in your ears, sound reaches you through the strings. When the fingers rest to the side of the head, the sound reaches your ear drum passing through air, where sound is slower.
Try this:
- You will need wire coat hanger and two pieces of string, each around 20 cm long.
- Tie a small loop at one end of each piece of string, big enough for your finger to fit inside.
- Tie the other ends of each piece of string to the corners of the coat hanger.
- Put your index fingers through the loops and allow the coat hanger to dangle upside down away from your body.
- Ask a friend to gently hit the coat hanger with a spoon or fork. What do you hear?
- Now, with the coat hanger still dangling from the strings around your fingers, bring your fingers to rest on the sides of your head near (but not in) your ears. You will need to bend forwards a bit so that the coat hanger can dangle freely.
- Ask your friend to hit the coat hanger again. What do you hear now?
In this classical experiment, which you can see repeated by Science Ireland here, your friend should hear the same sound no matter where your fingers are, but you might hear something different in the two cases. This has to do with how the sound reaches your ears in each case and, in particular, with the different speed of sound in air and in solids. When your fingers are touching your head next to your ears, the sound reaches your ear drum through the strings, at a faster speed. When your fingers are not touching your face, the sound reaches you through the air: not only it is slower, but energy is lost all around, so it sounds less loud. This is also why, in spaghetti-western movies, the hero can always hear an approaching train better by putting their ear on the rail tracks (do not do THIS at home!). A similar method is used by rail engineers (with a microphone attached to the track) for safety tests: if there is a fault along the track, the sound of approaching trains gets distorted.
A Sound Wave in Detail
We have learned that sound moves through a substance in waves, which consist of bunchings-up called compressions and stretchings-out called rarefactions. The movement of sound waves is tricky to draw, but we can make a graph to plot the pressure in a sound wave at a fixed instant in time (Figure 2), using a shape repeated over distance. Pressure is highest in a compression and lowest in a rarefaction, so our plot has alternating crests (peaks) and valleys (troughs). A single wave consists of one peak and one trough on our pressure plot.
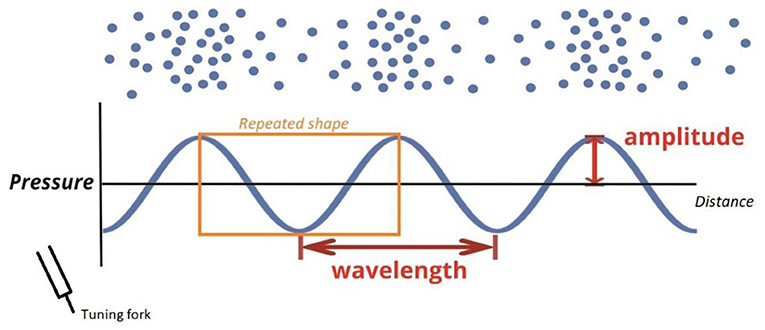
- Figure 2 - This figure represents what could be measured, at a fixed moment in time, when a single note is played (e.g., with a tuning fork).
- The tuning fork generates a pressure wave that travels at the speed of sound, causing a series of compressions and rarefactions of molecules. The pressure is highest in a compression, when the molecules are closer together, and lowest in a rarefaction, when they are mostly separated. The wave is represented by a repeated shape, whose height is the amplitude of the wave, measured in units of pressure (Pascal). The wavelength (measured in meters) is the distance needed for a point in the structure to repeat itself e.g., the distance between two peaks.
In Figure 2, the amplitude and the wavelength are marked—these are key features of sound waves. The amplitude is the height of the wave, measured from the top of a peak or the bottom of a trough. The wavelength is the length in meters from a fixed point on one repetition of the shape (e.g., its peak) to the same point on the next repetition.
Sound has another feature that does not show up on our plot—frequency. Frequency means “how often” and, in a fixed listening position, the frequency of a musical note tells us how many vibrations happen every second when that note is played. Frequency is measured in hertz (Hz) and, in the case of a single note (i.e., a single frequency, like in Figure 2), frequency and wavelength are related: waves with long wavelengths have low frequencies, and those with shorter wavelengths have higher frequencies.
Wavelengths for sound can be very large. As an example, the note usually used for tuning forks (440 Hz) has a wavelength on 78 cm (in air).
In general, however, a sound contains multiple frequencies (think about playing different notes simultaneously on a piano) and these determine the shape that repeats over distance in Figure 2. The frequencies in a sound often depend on how the sound is generated: using the same hammer to hit two bells of different size, for instance, may produce two very different frequencies.
Amplitude: How Loud Is a Sound?
One of the things that makes one sound different from another is the loudness, or volume, of the sound. If the volume of a movie is too low, you might not be able to hear what the characters are saying. If the music in your earbuds is too loud (at a high volume), it could damage your hearing!
The amplitude on our pressure graph tells us how loud the sound is. Loud sounds have strong vibrations, with high-pressure compressions and low-pressure rarefactions, so the greater the amplitude, the higher the volume and the louder the sound. Loudness is measured in decibels (dB). The decibel scale is logarithmic, which means it increases by multiplication rather than by addition. An increase of 10 dB means the sound is ten times as loud. But an increase of 20 dB is 10 × 10 = 100 times as loud. A 30-dB increase is 10 × 10 × 10 = 1,000 times louder. A whisper measures about 15 dB, normal talking about 60 dB, and a baby crying can reach as high as 110 dB! Sounds at 110 dB or more feel uncomfortably loud, and noises above about 130 dB are actually painful.
Try this:
- You will need a metal slinky, some tape and a plastic flowerpot.
- Hold a metal slinky at one end and let it hang to the floor.
- Lift a few coils so that they bunch together, then release them. What sound does it make?
- Tape the top of the slinky to the bottom of the plastic flowerpot (the opening of the flowerpot should face away from the slinky, as in Figure 3).
- Hold the flowerpot so that the slinky hangs down to the floor.
- Lift and release the coils as before. The noise made by the slinky alone is tinny and quiet, but with the flowerpot attached, it should be surprisingly loud!
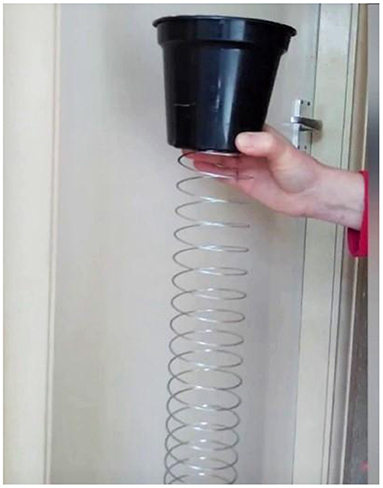
- Figure 3 - This experiment uses a slinky and a flowers pot to show how a container can increase the amplitude of a sound wave.
- First, the experimenter holds one end of the slinky and lets the other end fall. The sound produced in this way is very low in amplitude. Then, a flowers pot is attached to one end of the slinky (using tape), the experimenter holds the flowers pot and lets the other end of the slinky fall. The resulting sound, in this second case, is similar to a Star Wars laser blast (https://www.youtube.com/watch?v=3_JdIrQGKXc).
Why does this happen? On its own, the slinky has only a small vibrating surface acting on the air around it. The flowerpot, on the other hand, has a larger surface area and a large volume of air inside. When the two are attached together, the vibrations in the slinky make the flowerpot—and the air inside and outside it—vibrate. These vibrations build up and make the sound louder. We say the flowerpot amplifies the sound (makes it louder). You can do a similar experiment with your phone. Set it to play some music. Then, place it inside a dry mug to have the sound amplified.
In both cases, the size of the container determines which frequencies are amplified, and this could be used advantageously e.g., in metamaterials [1]. According to the ancient roman architect Vitruvius, for instance, small jars made of metal could be used in theaters to increase the volume of people singing: a theory that, according to some scientists, influenced the building of multiple European churches during the Middle Ages.
Pitch: How High or Low Is a Sound?
How high or low a noise sounds is called pitch. Pitch is related to the way a human perceives a sound’s frequency but related to how humans can hear it. Humans can hear frequencies between about 20 Hz (a low-pitched rumble, like thunder) and 20,000 Hz (a high-pitched whistle). In the standard way of tuning musical instruments, “middle C” (or C4) corresponds to 256 Hz, while the note A “above middle C” corresponds to the tone of a tuning fork (440 Hz). In music, going up one octave in pitch means doubling the frequency of the note. Interestingly, when we play two musical notes at the same time, those with frequencies in simple ratios (such as 20, 22, and 24 Hz) tend to sound more pleasing than combinations of notes with complex frequency ratios (such as 20, 21.5, and 37 Hz).
Many other animals can hear frequencies that humans cannot hear. Frequencies higher than humans can hear are called ultrasound, and those lower than our hearing-range are called infrasound. Echo-locating bats and dolphins use ultrasound to work out where objects are, and elephants use infrasound to communicate over long distances [2, 3].
Try this:
- You will need a balloon and a metal nut (the kind that screws onto a metal bolt).
- Place the nut inside the balloon.
- Blow up the balloon and tie a knot in the end.
- Hold the balloon near the knot and swirl it around so that the nut inside moves in circles.
You should hear a loud whirring noise as the edges and corners of the nut bounce off the inner surface of the balloon. This is because the nut is making the balloon vibrate and generating sound. Experiment by swirling the balloon at different speeds. What happens to the pitch of the sound as you alter the speed of the movement?
The pitch varies because the speed at which the nut is rotated affects the frequency of the sound produced. Higher speeds correspond to higher frequencies: if the number of rotations of the nut in a second doubles, the corresponding sound has increased in frequency by one octave. The size of the balloon is also important: for the same swirling movement, the velocity with which the nut rotates changes with the size of the balloon.
Who Studies Sound?
The study of sound is called acoustics, and the scientists and engineers who work on sound are called acousticians. Acousticians come from different specialties including biology, engineering, music, mathematics, physics, and psychology. They work on many aspects of sound, from environmental noise control, to understanding speech and language development. Others even study how to use sound waves to check the health of a baby before it is born. The one thing acousticians have in common is a fascination with sound. We hope you share this fascination and enjoy these noisy at-home experiments. Maybe you will inspire a future acoustician or even become one yourself!
Glossary
Compression: ↑ The part of a wave in which the molecules are closest together.
Rarefaction: ↑ The part of a wave in which the particles are farthest apart.
Amplitude: ↑ The height of a wave, measured from its position to the top of a peak or the bottom of a trough.
Wavelength: ↑ The length of a wave, obtained at a fixed instant in time by measuring the distance between a point on a wave and its next repetition.
Frequency: ↑ The number of waves that pass a fixed point in 1 s. Measured in hertz (Hz).
Volume: ↑ In this article, we defined “volume” the amplitude of a sound wave expressed in decibel.
Decibels: ↑ Decibels are a relative scale for amplitude, so the volume measures how many times the amplitude of a pressure wave is bigger than a reference pressure (For sounds traveling in air, this reference is 0.00002 Pascal (20 micro Pascal), which is the minimum pressure humans can hear a note at 1,000 Hz).
Pitch: ↑ According to Britannica, it is “the position of a single sound in the complete range of sounds”. It is a quantity related to the frequency of a sound, but on a relative scale.
Ultrasound: ↑ Sounds at frequencies too high for humans to hear them. This means typically above 20,000 Hz. Animals like bats use ultrasound to fly and hunt.
Infrasound: ↑ Sound sat frequencies too low for humans to hear them. Typically this means below 20 Hz. Infrasounds are created by very large objects, like wind turbines.
Acoustics: ↑ According to Britannica, it is “the science concerned with the production, control, transmission, reception, and effects of sound”. Acoustics covers also frequencies beyond the range humans can hear.
Acknowledgments
GM acknowledges funding from UK Research and Innovation through grant EP/S001832/1 AURORA: Controlling sound like we do with light.
Conflict of Interest
The authors declare that the research was conducted in the absence of any commercial or financial relationships that could be construed as a potential conflict of interest.
References
[1] ↑ Li, J., Wen, X., Sheng, P. 2021. Acoustic metamaterials. J. Appl. Phys. 129:171103. doi: 10.1063/5.0046878
[2] ↑ Simmons, J. A., Houser, D., and Kloepper, L. 2014. “Localization and classification of targets by echolocating bats and dolphins,” in Biosonar, eds A. Surlykke, P. E. Nachtigall, R. R. Fay, and A. N. Popper (New York, NY: Springer New York). p. 169–193.
[3] ↑ Garstang, M. 2010. “Chapter 3.2 - Elephant infrasounds: long-range communication,” in Handbook of Behavioral Neuroscience, Vol. 19, ed S. M. Brudzynski (Elsevier). p. 57–67.